Fig. 19.1
IOM techniques can be divided into four groups depending on susceptibility to the effects of muscle relaxants and to the effects of halogenated anesthetics
The second consideration is how difficult it is to conduct IOM in an individual patient. This is usually a consequence of either immaturity at very young ages or as a consequence of neural pathology, especially in the older patient. This is depicted in Fig. 19.2. Adult patients may have nervous system deficits from age and from neural morbidity such as myelopathy, vascular disease, diabetes, and other primary central nervous system (CNS) pathology. In both of these groups the responses may be difficult to acquire and the anesthesia effects may be more profound. In addition, patients with chronic opioid use can also provide challenges by limiting the effectiveness of opioids, which are usually key agents in the anesthetic. Finally, the chosen anesthetic must address the specific medical comorbidities of the patient and those management aspects necessary for the conduct of the surgery.
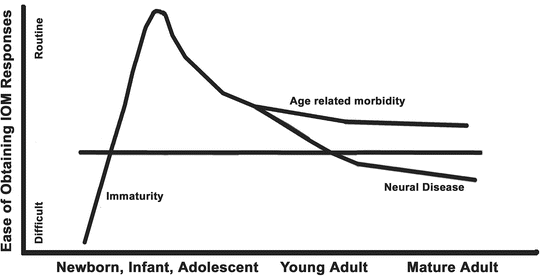
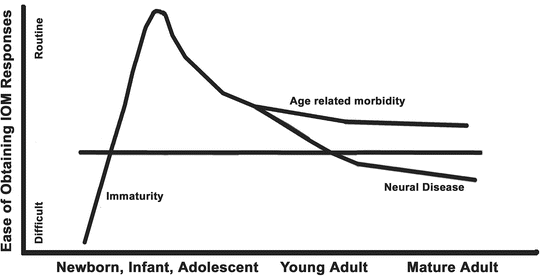
Fig. 19.2
The ease of recording evoked responses varies with patient age, neural immaturity at young ages, and the presence of neural pathology
Mechanism of Drug Action
It is currently believed that although anesthetic agents must have properties of lipid solubility to penetrate into the nervous system, the action of these drugs is primarily mediated through changes in synaptic function by their interaction with specific receptors [1–10]. Differences between agents depend on each one’s profile of action on different synaptic receptors, the varying location of those receptors, and the specific subtypes of these receptors involved in their action.
For example, many of these agents increase the inhibitory activity in the major inhibitory synapse by facilitating the effect of γ-amino-butyric acid (GABA) by action at the GABAa receptor [10]. Another common drug effect is inhibition of the major excitatory synapse at the N-methyl-d-aspartate (NMDA) receptor [10]. Other synaptic targets include neuronal acetylcholine (nACh), μ opioid receptor, central α2 receptors, potassium, calcium receptors, and the glycine channels (the major inhibitory synapse in the spinal cord) [10]. Muscle relaxation is mediated through acetylcholine receptors located at the neuromuscular junction (mACh) [10]. The combination of effects at these receptors is what gives rise to the effects of general anesthesia as well as the alterations in the electroencephalogram (EEG) and evoked potentials.
Anesthetic Effects on the Electroencephalogram
General Effects
Because the EEG is produced by cortical synapses, anesthetic agents alter EEG monitoring [11] (see Chap. 10, “EEG Monitoring”). Although differences between individuals and anesthetics occur, most anesthetics produce a typical sequence of events with increasing drug levels [12, 13] (Fig. 19.3). At induction, mild activation is seen with rhythmic beta activity replacing the 8- to 10-Hz α rhythm seen in the parietal and superior temporal cortex. With loss of consciousness there is a marked drop in high frequency activity (25–50 Hz gamma-band), an increase in slower frequency theta and delta activity, and a shift in power to the frontal regions. In addition, there is an increase in synchrony of the EEG with variations in activity between regions being reduced including uncoupling of the interaction between frontal and parietal regions and across the midline [13]. As the anesthetic drug effect increases there is a gradual slowing of the frequency and gradual reduction in amplitude and power. Then periods of electrical silence are interspersed with periods of activity (burst suppression) and finally complete electrical silence.
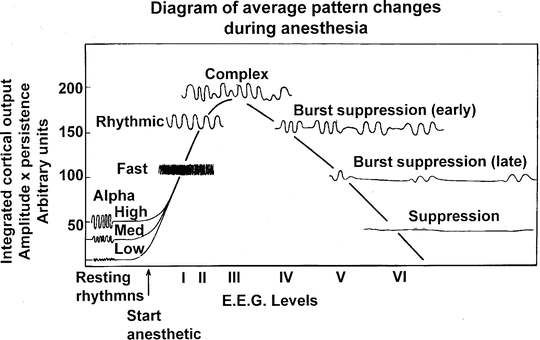
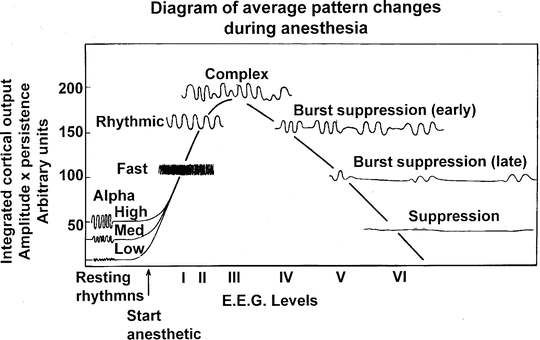
Fig. 19.3
Typical EEG changes with progressively increasing levels of inhalational anesthesia . As shown, the amplitude increases as the variability of the EEG is reduced to synchronous activity in the 8- to 12-Hz range. As anesthesia deepens, the amplitude and frequency decrease, giving way to burst suppression and finally electrocerebral silence. (From Stockard and Bickford [12]; with permission)
The reduction in synaptic activity at burst suppression and electrical silence is associated with a reduction in the associated metabolic activity of approximately 50 % (Fig. 19.4). This depressant effect has been used for the treatment of status epilepticus and for intentional metabolic suppression to improve the balance of nutrient supply and demand such as during surgery in which ischemic injury may occur (e.g., intracranial vascular surgery, cardiac surgery, and carotid surgery). Because burst suppression is associated with near maximal metabolic suppression, this can be used as an end point to monitor the drug administration for metabolic depression.
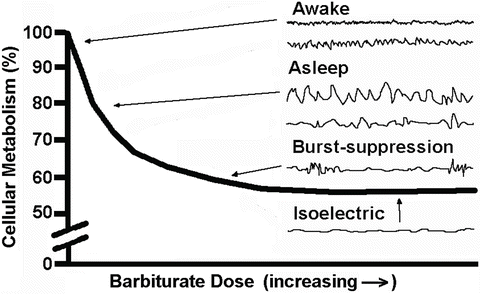
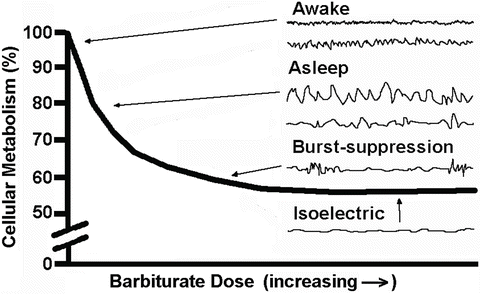
Fig. 19.4
Depiction of the progressive decrease in cerebral metabolism as barbiturate dose is increased until 40–50 % remains. Also shown are typical EEG tracings with increasing doses. Of note is that electrocerebral silence is associated with maximal metabolic suppression and near maximal suppression occurs when burst suppression is seen on the EEG
Specific Drugs and the EEG
Halogenated Agents
The halogenated anesthetic agents produce the typical depression pattern of Fig. 19.3, producing burst suppression at about 1.5 or higher minimum alveolar concentration (MAC) (the MAC of an inhalational agent is the minimal alveolar concentration of the agent where 50 % of the subjects do not move in response to a painful stimulus) [14]. With increasing age, the brain becomes increasingly sensitive to anesthetics and burst suppression is produced at lower concentrations. Halothane does not follow the typical dose effect of the other agents; it does not readily cause burst suppression but rather a gradual decrease in amplitude with widespread α activity (8–14 Hz) [15].
Not all anesthetic agents produce this general pattern; some can activate the EEG resulting in increased amplitude and frequency with a few ultimately producing epileptiform activity [11]. For example, enflurane and sevoflurane produce EEG changes similar to those of isoflurane but have been associated with increased EEG activity and seizures in some circumstances. With hyperventilation they can produce epileptiform spikes or even electrographic seizures. With these seizures, anesthetic depression of the spinal cord may prevent associated muscular activity (see “Immobility” later) [16]. Sevoflurane can also induce epileptiform activity in patients with epilepsy [17]. Rapid induction with sevoflurane, particularly with hyperventilation and prolonged anesthesia with high concentrations, can produce generalized or focal seizures (even in healthy subjects).
Nitrous Oxide
When used alone, nitrous oxide (N2O) decreases the frequency and amplitude of α rhythms seen in a restful awake state. It then produces a frontally dominant high frequency (>30 Hz) activity, which coincides with analgesia and depressed consciousness. When combined with halogenated inhalational agents, it can be additive or antagonistic depending on the circumstances and the neurophysiological measure used. Thus, N2O may be “context sensitive.” For example, N2O has proconvulsant properties or can suppress epileptic spike activity during electrocorticography (ECoG) depending on the other agents used.
Propofol
Propofol produces the typical EEG anesthetic depression described earlier, including burst suppression and electrical silence at higher doses [18]. As such, propofol has been used for the treatment of status epilepticus and to produce metabolic depression. Despite its seizure-suppressive activity, propofol has become a popular agent for conscious sedation during surgery to identify and ablate seizure foci because its rapid metabolism allows it to be eliminated when awake testing and ECoG is desired.
Etomidate
Etomidate produces the typical EEG anesthetic depression pattern described earlier. Like propofol, it has been used to produce EEG silence and metabolic depression and should be avoided at larger doses during cortical mapping for seizure foci. Different than propofol, at low doses (0.1 mg/kg) it may enhance the detection of seizure foci by evoking native epileptic seizures in patients [19].
Barbiturates
Barbiturates follow the typical pattern of EEG progression described earlier leading to electrical silence. They are the classic agents to produce metabolic depression (“barbiturate coma”). Not typical of all barbiturates, low-dose methohexital (0.5 mg/kg) has been used like etomidate to enhance epileptic spike activity during electrocorticography (ECoG) in surgery to identify seizure foci [19].
Benzodiazepines
Most benzodiazepines produce the typical EEG anesthetic depression pattern but have a less profound effect on the EEG, usually generalized slowing into the theta and delta range at high doses without progressing to burst suppression. They have potent anticonvulsant effects such that they should be avoided if cortical mapping for seizure foci is planned. Midazolam can produce burst suppression and this is used in treatment of status epilepticus.
Dexmedetomidine
Droperidol
Droperidol has little effect on the EEG when used alone; however, it lowers seizure threshold. It does not appear to produce neuroexcitatory phenomena or induce seizures in epileptic patients. When combined with fentanyl (“neurolept anesthesia”), droperidol increases EEG α activity at low doses. At higher doses, it produces high-amplitude beta and delta activity.
Opioids
Opioids do not appear to produce an initial excitement phase in the EEG and do not produce burst suppression or electrical silence. They do produce a dose-related decrease in frequency of the EEG maintaining amplitude with activity in the delta range. They are frequently used during ECoG in surgery for seizure focus ablation. Some clinicians have found alfentanil useful in enhancing epileptic spikes [13].
Ketamine
Ketamine produces initial suppression of awake α rhythms, and then induces high-amplitude theta activity in the EEG, with an accompanying increase in beta activity [21]. At large doses, polymorphic delta with interspersed beta is reported [22]. Ketamine is thus an excitatory agent producing heightened synaptic function and increased motor tone and muscle movement. Seizures have not generally been observed and it is usually anticonvulsive, but it has been reported to provoke seizure activity in persons with epilepsy but not in normal subjects [23, 24].
Anesthesia Selection and EEG
The choice of anesthetic agents depends on the specific application of the EEG [25]. When monitoring for cerebral ischemia, a choice of drugs that keep the EEG active is desired. For the termination of sustained seizure activity or intentional depression of cerebral metabolic activity, intravenous agents (e.g., propofol) have been used. General anesthesia with the halogenated agents has also been used to treat status epilepticus. For the recording of seizure foci during awake craniotomy for the purposes of seizure focus ablation, infusions of short-acting agents have been used (with local anesthesia for the craniotomy and placement of a Mayfield head holder) (see Chaps. 18, “Anesthesia for Awake Neurosurgery”, and 45, “Epilepsy and Seizures: OR and ICU Applications of EEG”). When seizure focus ablation is conducted under general anesthesia, agents that are minimally depressant should be used during ECoG.
Anesthetic Effects on Evoked Potentials
Consistent with a synaptic mediated effect, the effect of anesthetic agents on cortical evoked responses parallels their effect on the EEG. This has been shown by Winters for the midlatency cortical auditory (MLAEP) response [26]. Similar to the EEG, most anesthetic agents cause a progressive depression of the cortical evoked responses, although some agents are excitatory (e.g., etomidate, ketamine). Also like the effects on the EEG, some agents (e.g., opioids) cause much fewer dramatic changes, making them desirable during IOM. Because general anesthetics primarily affect synaptic function rather than neuronal conduction, the majority of anesthetic effects are amplitude changes with smaller changes in latency [27]. For a more in-depth discussion of using the EEG to assess sedation and anesthesia, see Chap. 11, “Clinical Application of Raw and Processed EEG.”
Effect Based on Location of Synapses
In general, for each IOM modality, the effects of anesthetic agents can be somewhat predicted by noting the location of synapses within the neural pathway. For example, the somatosensory evoked potential (SSEP) pathway has no synapses until the cervicomedullary junction (near the nucleatus cuneatus and nucleatus gracilis) (see Chap. 1, “Somatosensory Evoked Potentials”). As such, recordings of the SSEP response in the peripheral nerve (e.g., popliteal fossa, Erb’s point), along the spinal cord (e.g., epidural), or the subcortical response recorded over the cervical spine show minimal anesthetic effects. Major anesthetic effects are seen at or above the second synapse located in the thalamus (i.e., the cerebral cortex response). These effects are shown in Fig. 19.5 with isoflurane and are typical for most anesthetics, which are depressant on the EEG and evoked potentials.
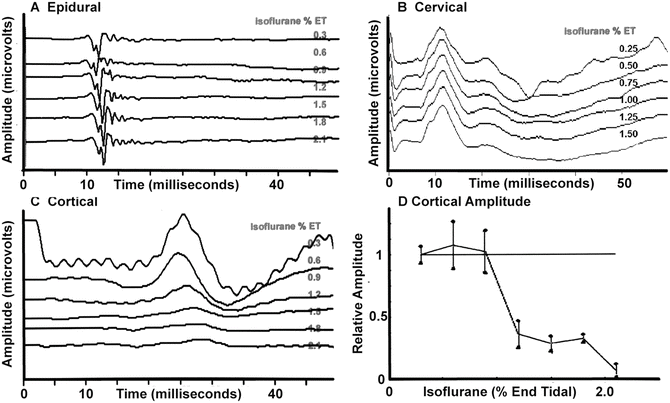
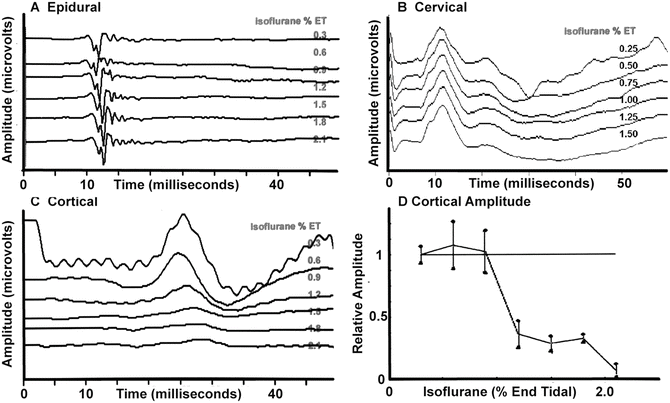
Fig. 19.5
Changes in lower extremity somatosensory evoked potentials recorded at several locations with increasing concentrations of isoflurane in the baboon. (a) Recordings from the epidural space that indicate minimal effects. (b) Minimal effects in the response recorded over the cervical spine. (c) A prominent effect on the response recorded over the somatosensory cortex. (d) A plot of the amplitude of the cortical response showing a nonlinear amplitude reduction as the isoflurane concentration is increased
For the auditory brainstem response (ABR), few synapses are involved in the brainstem such that anesthetic effects are minimal (see Chap. 3, “Auditory Evoked Potentials”). Because the cortical midlatency response (MLAEP) is heavily dependent on synaptic function, the anesthetic impact is far more substantial.
Motor evoked potentials (MEP) are susceptible to anesthetic agents at several synaptic locations (see Chap. 2, “Transcranial Motor Evoked Potentials”). The first location is in the motor cortex where anesthetic depression of internuncial neurons reduces production of “I” waves. Because the “D” waves are the result of direct pyramidal cell stimulation, they are relatively unaffected by anesthetic agents [28, 29]. This maintenance of D waves and loss of I waves with increasing isoflurane is shown in the baboon in Fig. 19.6.
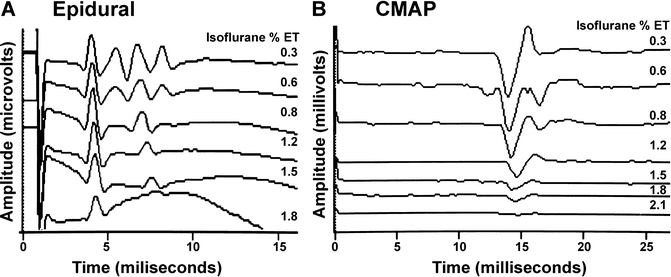
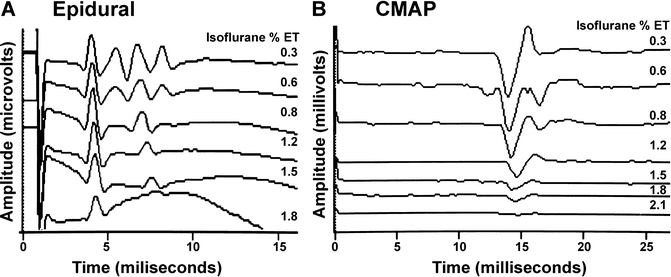
Fig. 19.6
Changes in transcranial motor evoked potentials recorded in the epidural space (a) and in the myogenic response (compound muscle action potentials; CMAP) in the hand (b) in the baboon. Shown is the maintenance of the single D wave (“D”) and loss of the multiple I waves (“I”) in the epidural recording and loss of the myogenic response with increasing concentrations of isoflurane
The second location is internuncial synapses within the spinal cord. The anesthetic impact on any given pathway likely depends on the number of these synapses involved. The descending motor tracts follow through lateral and medial pathways with the more distal muscles (i.e., hand and foot) served by the lateral pathway. Because this lateral pathway has fewer synapses, myogenic MEP in these distal muscles (hands and feet) may be slightly less affected by anesthetics than the truncal muscles served by the medial pathway.
Anesthetic action at the anterior horn cell may also impair the generation of a muscle response through synaptic depression. Since the D and I waves must summate temporally to activate the anterior horn cell, the loss of I waves increases the difficulty of obtaining a myogenic response (compound muscle action potential [CMAP] ) with a single transcranial pulse stimulation [30, 31]. In addition, other descending tracts (descending suprasegmental systems [corticospinal, rubrospinal, vestibulospinal, and reticulospinal systems] and propriospinal systems) influence the excitability of the anterior horn cell such that anesthetic effect in these pathways could result in additional effect on the myogenic MEP.
This need for temporal summation and the loss of I waves explains some of the success of multipulse stimulation technique, which produces a volley of D waves [32, 33]. At higher anesthetic doses, an even more profound synaptic block at the anterior horn cell may prohibit synaptic transmission, regardless of the composition of the descending spinal cord volley of activity. The spinal cord has been suggested as the location of the most prominent anesthetic effect on myogenic MEP responses [34].
One additional synapse that may be affected by anesthesia in the motor pathway is the neuromuscular junction. Fortunately, with the exception of neuromuscular blocking agents, anesthetic drugs have little effect at the neuromuscular junction.
Effect Based on Anesthesia Goals
Additional insight into the action of anesthetics on evoked responses is given by examining the neural mechanisms of anesthetics that result in the behavioral goals of amnesia, unconsciousness, lack of movement in response to noxious stimuli (immobility), and blocking of noxious sensory stimuli (antinociception).
Amnesia
Amnesia is thought to be one of the most potent effects of many general anesthetics. It is thought that this involves anesthetic action at the GABAa receptor (with additional contributions at the NMDA and nACh synapses) in the hippocampus, amygdala, and entorhinal and/or perirhinal cortex. Fortunately, anesthetic agents usually block memory formation at or below drug levels that produce unconsciousness [10, 35].
Unconsciousness
Consciousness is likely mediated by a neural network involving the thalamocortical, corticothalamic, and reticulothalamic neurons [36]. Anesthetic action producing unconsciousness is thought to be mediated primarily through the GABAa and central α2 receptors, with additional contributions through the NMDA, potassium channels, and nACh receptors. In general, most anesthetics produce unconsciousness through mechanisms including (1) reducing arousal stimuli to the cerebral cortex by actions at the brainstem (midbrain reticular formation), (2) interfering with cortical processing of information, and (3) blocking sensory information from being received by the cortex through actions at the thalamus (“thalamic gating”) and at the dorsal horn of the spinal cord [37]. This suggests that the major impact of drugs producing unconsciousness will be depression of the cortical sensory responses (SSEP, MLAEP, and visual evoked potential [VEP]).
It appears that the neural mechanisms producing unconsciousness are nonlinear, similar to an “on–off” system with an abrupt loss of consciousness over a narrow increase of anesthetic concentration [10]. This has been termed a “consciousness switch” and depends on the interaction of two sets of nuclei [36]. One set promotes consciousness (Laterodorsal Tegmental Nucleus, Pedunculopontine Nucleus, Locus Coeruleus, Dorsal Raphe, Ventral Periaqueductal Gray, and Tuberomammillary Nucleus) and the other promotes unconsciousness (Ventrolateral Preoptic Nucleus and Median Preoptic Nucleus). This suggests the anesthetic impact on cortical sensory responses may also be on–off with the major amplitude change occurring over a narrow anesthetic dosage range. The “threshold” for this switch appears to vary with patients as well as with anesthetic agents and may define the ability to use anesthetic agents at concentrations near those that produce unconsciousness.
Immobility
With unconsciousness, immobility (lack of movement to noxious sensory stimuli) is the consequence of anesthetic action interrupting the reflex pathway in the spinal cord. This effect is used to characterize the anesthetic potency of inhalational agents termed “MAC” suggesting halogenated agents will be effective in blocking movement at MAC levels [9]. The major anesthetic effect responsible for this is action of halogenated agents which is thought to be mediated through the spinal cord glycine channels with some contribution at GABAa [38] (which is thought to be the mechanism of etomidate and propofol [35, 39]).
The parts of this reflex pathway include afferent sensory stimuli, which can be effected in the periphery and in the dorsal horn of the spinal cord. The second part is the neural pathways within the spinal cord. This includes interneurons in the reflex as well as the anterior horn cell. The last component is the efferent pathway from the anterior horn to the muscle and includes transmission through the neuromuscular junction. Hence, immobility is a consequence of anesthetic action blocking sensory afferents, reflex pathways in the spinal cord, and motor efferents. In addition, anesthetic action at the neuromuscular junction will affect movement. Although the reflex pathways can be modulated by descending influences, evidence suggests that immobility is primarily a spinal cord action and is largely independent of drug effect at the brainstem and cortex [40].
The net effect of this pathway is that anesthetic effects on the different parts contribute to immobility. Thus, a balance can be achieved with agents that reduce afferent sensory stimuli, agents that interfere with spinal cord transmission, and agents that reduce the efferent activity. This may allow immobility to be achieved with doses of these agents that allow IOM recording when otherwise higher doses of each agent would be needed and prevent recording.
Antinociception
In addition to blocking sensory transmission to the brain (such as at the thalamus mentioned earlier), the blocking of noxious stimuli (“antinociception”) by drug action also occurs throughout the brain and spinal cord. In the spinal cord, sensory stimuli are modulated in the dorsal horn by NMDA and opioid synapses. Descending influences from the midbrain and brainstem also exert an inhibitory or excitatory effect on the dorsal horn transmission. Alpha2 agonists mediate some of the antinociception either directly, modulating descending influences, or by enhancing opioid action [41]. Opioids, norepinephrine, and serotonin play a role in these descending inhibitory pathways [42]. As mentioned earlier, anesthetic effects that reduce sensory transmission through the thalamus and alter cortical processing of sensory information also contribute to antinociception.
Specific Anesthesia Drugs
The differences between drugs are likely a consequence of differing profiles on receptor types (e.g., GABA, NMDA), differing location of drug action (i.e., pre- or postsynaptic effects), the spectrum of effects on individual subtypes of these receptors, and the anatomic distribution of the receptors and subtypes. Hence, the choice of an anesthetic technique becomes a balance of attempting to use concentrations of agents that are supportive of monitoring while accomplishing behavioral goals and the needs of the procedure. Some agents are more supportive of the monitoring techniques and are usually favored.
Halogenated Inhalational Agents
The most prominent anesthetic effects on evoked responses during clinical anesthesia are those of the halogenated inhalational agents (e.g., isoflurane, sevoflurane, desflurane), which makes them most difficult to use with some IOM techniques (notably myogenic MEP). These agents have a broad action on neural structures and are very effective in producing unconsciousness and amnesia through their action at the GABAa receptor. They are also very effective in producing immobility through their effect at the spinal cord glycine receptor. In addition, they contribute to antinociception via actions at the NMDA, nACh, and potassium channels.
For the SSEP, the predominant anesthetic effect is on responses generated above the level of the thalamus [43, 44] (Fig. 19.5). The blocking of sensory transmission at the thalamus is shown by the reduction of spontaneous and evoked output of the thalamic relay nuclei starting at 0.3–0.5 MAC [1, 37, 44]. This explains why cortical sensory evoked responses can often not be recorded with concentrations above 0.5–1 MAC [45, 46]. Above this level the inhalational agents produce marked effects in a nonlinear, on–off fashion consistent with anesthetic action on consciousness. Hence, the inhalational agents must be limited in most patients to 0.5–1 MAC when monitoring the cortical SSEP. The threshold of the ability to record varies between patients and the presence of neural pathology. In some patients, such as those with very poor responses (e.g., neural degeneration), the halogenated agents may need to be severely restricted (e.g., <0.5 MAC) or not used at all if responses are to be obtained.
Fortunately, responses recorded at peripheral nerves, over the cervical spinal cord, and from electrodes in the epidural space or spinal canal can usually be recorded with only minimal anesthetic effect [47]. Similarly, the ABR can also be recorded without major anesthetic effect. However, the cortical auditory response (MLAEP) is similar to the cortical SSEP with marked anesthetic effect.
The halogenated anesthetic agents decrease VEP amplitude and increase latency [48–50]. Studies of the a and b wave of the electroretinogram show that halogenated agents (and sedatives) decrease amplitude with some agents increasing the response latencies [51, 52]. One study noted with induction with sevoflurane the responses were lost [53].
The effect of the halogenated agents on the spinal reflex mediating immobility is used to determine MAC and is a prominent action of the halogenated agents. Of note, the concentration–effect curve is rather steep with changes in the spinal cord reflex pathway occurring over a relatively narrow concentration range [54]. This may explain the difficulty of finding a suitable anesthetic concentration, which allows recording of myogenic MEP responses.
Because of the anesthetic effect at the spinal cord, the myogenic response of the MEP is the most challenging to record with the halogenated inhalational agents and may require them to not be used (Fig. 19.6). When recordable, the major anesthetic effect may occur at low concentrations (e.g., <0.2–0.5 % isoflurane) with loss of responses above 0.3–0.5 MAC [55–57]. Consistent with the depression of reflex movement by a spinal action of halogenated anesthetics, these agents also depress the Hoffmann reflex (H-reflex) [58, 59]. Studies suggest that the myogenic MEP is depressed more than the H-reflex (50 % vs. 22 % with sevoflurane) [59, 60].
Several recent studies have shown that in some patients, myogenic responses can be recorded with 0.5 MAC of desflurane or sevoflurane [61–64]. In these cases, the amplitude of the MEP is reduced compared with TIVA but monitoring appears to be conducted successfully. In a few patients the MEP could not be recorded until the halogenated agent was eliminated and TIVA used; in one patient it was never recorded. This suggests that the use of 0.5 MAC of a halogenated agent should be reserved for patients with robust responses (i.e., minimal neural pathology) and when used, preparations for converting to TIVA be available when responses cannot be acquired at baseline. At present, it is unclear whether desflurane or sevoflurane is less depressant at these doses [63].
Since the D wave is usually unaffected by anesthesia, it can usually be recorded in the epidural space or spinal canal at anesthetic concentrations of halogenated agents.
Nitrous Oxide
As a nonhalogenated inhalational agent , N2O is different from the halogenated agents; N2O is particularly effective in antinociception due to its action at the NMDA receptor with additional contributions at the μ opioid, nACh, and potassium channels. In addition, it contributes to unconsciousness and amnesia through minor actions at the GABAa and central α2 receptors and contributes to immobility through minor actions at the glycine receptor [65].
The effects of nitrous oxide on the SSEP are similar to the halogenated agents, although the effects vary with the other anesthetic agents being employed. When used alone, nitrous oxide tends to produce graded amplitude and latency changes in a dose-dependent manner [66, 67], with minor or no changes in subcortical responses [47]. Nitrous oxide is a more potent depressant of the P15–N20 SSEP response than isoflurane when compared on a MAC basis [68]. Because nitrous oxide is very insoluble, the changes can occur rapidly. Nitrous oxide reduces VEP amplitude with increase in latency at higher doses. When nitrous oxide is added to a halogenated agent, the VEP is lost.
When added to halogenated anesthetics, nitrous oxide may cause additional changes in latency and amplitude [47] or have no apparent additive effect [45]. Studies of equal MAC mixtures of isoflurane and nitrous oxide have demonstrated that the mixture has a more potent effect on cortical SSEP than would be predicted by adding the individual effects of each agent, suggesting a synergism from different mechanisms of action [69]. In cases in which nitrous oxide is added to intravenous agents, amplitude changes predominate, without latency change [70–72].
Studies with MEP [73, 74] show that N2O produces depression of myogenic responses similar to the halogenated agents [56, 72, 74]. When compared at equal MAC concentrations, nitrous oxide produces more profound changes in myogenic MEP than any other inhalational anesthetic agent [68, 75]. Similar to low doses of halogenated agents, myogenic MEP can be recorded in some patients when N2O is used in concentrations below 50–60 % [74]. As with other modalities, the effect of N2O is dependent on the other anesthetics used with marked depression when N2O is combined with a halogenated agent [76, 77]. Like the halogenated agents, the effects on the epidurally recorded MEP are minimal.
Intravenous Agents
Sedative-Hypnotics: Propofol
When the concentration of halogenated agents must be restricted, intravenous sedative hypnotics are usually employed to achieve amnesia and unconsciousness. Of the agents available, propofol is currently the most commonly utilized agent. Propofol has potent effects on unconsciousness and amnesia via actions at the GABAa receptor. This action at the GABAa and minor effects at the glycine receptor contribute to immobility during anesthesia. Finally, it makes minor contributions to antinociception through actions at the glycine and nACh receptors.
Propofol induction produces amplitude depression in cortical SSEP, VEP, and MLAEP with rapid recovery after cessation of a short infusion (actual time for longer infusions is dependent on the duration of the infusion, a consequence of the context-sensitive half-time) [78, 79]. Propofol does not enhance cortical responses. When the SSEP is recorded in the epidural space, propofol has no significant effect. At sedative doses, the SSEP is affected at the cortex only. At higher doses (hypnosis), the thalamic response is also affected [80]. At anesthetic doses, the cortical SSEP response amplitude is reduced and is lost at higher doses [56, 81–83].
Propofol induction produces amplitude depression in cortical responses and myogenic MEP with minimal effects on the epidurally recorded D wave with rapid recovery after cessation of infusion [34, 56, 81–83]. Similar to the halogenated agents, I waves are lost during propofol anesthesia consistent with synaptic effects on the brain similar to the depression of the EEG. When propofol is studied without other agents (and without surgical stimulation), MEPs can usually be recorded at burst suppression, although the amplitude is lower than at lighter levels [84].
Different from the steep concentration–immobility effect curve of the halogenated agents, the dose–response curve on immobility is substantially flattened with changes occurring over a wider concentration range [54]. This suggests that it is more likely to identify a concentration where propofol provides adequate anesthetic effect and acceptable depression of the H-reflex and myogenic MEP than with a halogenated agent [58, 81–83, 85].
At doses consistent with adequate anesthesia, patients with neurological compromise and poor responses may not have recordable myogenic MEP with propofol [54]. The same situation arises if higher doses of propofol are needed, such as with chronic GABAa acting drug usage (e.g., ethanol, benzodiazepines) or when an increased effect of propofol is needed to accomplish immobility when opioid tolerance produces poor antinociception [54]. When higher propofol doses appear to be needed, some TIVA methods have used ketamine or lidocaine to provide additional drug effect so that the dose of propofol can be reduced into an acceptable range [86].
Etomidate
An alternative sedative-hypnotic is etomidate, which also has potent cortical effects on unconsciousness and amnesia via actions at the GABAa receptor. It contributes to immobility via actions at the GABAa and glycine receptors, and has some minor contributions to antinociception through actions on the potassium channels. Of note, its use in TIVA has been reduced in many centers due to concerns of adrenal suppression and worsened outcome, especially in sepsis [87, 88].
At low doses , etomidate also enhances the amplitude of the cortical SSEP [89–94]. Fortunately, the enhancing activity occurs at doses that are consistent with the desired degree of unconsciousness and amnesia needed for TIVA. This amplitude increase appears coincident with the myoclonus seen with the drug, suggesting a heightened cortical excitability (however, no evidence of seizure activity was seen) [95, 96]. A sustained increase with constant drug infusion has been used to enhance cortical SSEP monitoring that was otherwise not recordable [89–94]. A cat study suggests that the location of enhancement is cortical [96], which is consistent with clinical studies showing enhancement of cortical responses with no enhancement in subcortical responses [90]. Higher doses of etomidate cause depression of the evoked responses (similar to the EEG) suggesting a biphasic effect (enhancement followed by depression).
Studies with myogenic MEP have suggested that etomidate is an excellent agent for induction and monitoring of this modality. Of several intravenous agents studied, etomidate had the least degree of amplitude depression after induction doses or with continual intravenous infusion [81, 82, 97–100]. At low doses, etomidate also enhances the myogenic MEP amplitude [89–94, 100]. This appears coincident with the myoclonus seen with the drug, suggesting a heightened cortical excitability [95, 96]. It is interesting that etomidate also increases the H-reflex, suggesting a change in α motor neuron excitability in the spinal cord [101]. This effect may be contributing to the enhancement of myogenic responses of MEP [81, 82, 97–100].
Benzodiazepines
Benzodiazepines , notably midazolam, also act at the GABAa receptor and have been advocated as supplements to TIVA because of amnestic properties and the ability to reduce the chance of hallucinogenic activity with ketamine. Midazolam, in doses consistent with induction of anesthesia and in the absence of other agents, produces a mild depression of the cortical SSEP [81, 93, 95, 102–105]. It has also been used in TIVA, prior to the introduction of propofol, with conditions suitable for anesthesia and cortical SSEP monitoring [90]. As such, when myogenic MEP is monitored, small doses for premedication or occasional supplementation during anesthesia usually allows myogenic MEP recording. Unfortunately, benzodiazepines (notably midazolam) produce marked acute and prolonged depression of myogenic MEP at larger doses [81, 82, 93, 95, 102–105].
In addition to possible cortical locations for the benzodiazepine effect, an effect at the spinal cord has been described as antinociceptive through actions at the GABA receptors in lamina I and II in the dorsal horn [106, 107]. This drug action is thought to be responsible for the depression of the H-reflex by diazepam [106, 107].
Dexmedetomidine
Another agent used to promote unconsciousness is dexmedetomidine , which acts as a central, selective α2 adrenoreceptor agonist drug. Dexmedetomidine has been shown to reduce the amount of propofol, opioids, and halogenated inhalational agents needed during anesthesia [108]. Of note, it does not produce amnesia. Side effects of hypotension and bradycardia relate to its sympatholytic properties and limit the drug to a role as a supplement to other anesthetic agents. The effects on SSEP recordings are minimal, possibly due to selective brainstem action at the locus coeruleus such that thalamic blocking is minimal. But, as with propofol, higher blood levels of dexmedetomidine inhibit MEP monitoring. When used as a supplement to isoflurane or a propofol-fentanyl-nitrous oxide anesthetic, no additional depression to the cortical SSEP is seen [109].
Barbiturates
In general, the effects of barbiturates on the cortical SSEP are very small [113, 114], but the effect of thiopental can be rather profound and long lasting on the myogenic MEP at higher doses [77, 82, 97]. Methohexital has been used as a substitute for propofol in TIVA with acceptable cortical SSEP and myogenic MEP monitoring [113–115].
Intravenous Antinociception: Opioids
To achieve all four anesthetic goals using intravenous anesthetics, opioids or ketamine is usually used to produce antinociception. Of note, these agents also help with immobility due to actions on the afferent portion of the reflex. Like its effects on the EEG, opioid effects on SSEP and MEP are minimal, especially on spinal or subcortical recordings [28, 29, 56, 73, 81, 82, 98, 103, 118–135]. The effects on the SSEP are reversed with naloxone, suggesting that the effect is mediated by the μ receptor [136, 137]. Some transient depression of amplitude and increases of latency in cortical responses can be seen with bolus doses and occasional loss of late cortical peaks (over 100 ms) at higher doses [81, 97, 116]. The spinal application of morphine or fentanyl produces minimal changes in the cortical SSEP [70, 76]. Several studies have shown a minimal depressant effect of clinical doses of opioids on the myogenic MEP [28, 29, 56, 73, 81, 82, 98, 103, 118–135].
Opioids can potentiate the effects of propofol. In addition, bolus doses of these agents will produce transient depression of responses and higher concentrations can persistently produce significant depression. As such, the delivery of opioid by infusion is important during anesthetic maintenance similar to the use of infusions of other agents [138]. Opioids appear to have minimal effects on the VEP, except that pupil constriction can reduce the effectiveness of the stimulation reducing amplitude and bolus doses can transiently reduce the amplitude [137, 139].
Ketamine
As an alternative or supplement to the opioids (particularly when the patient is opioid tolerant), ketamine has very potent antinociceptive actions via its action at the NMDA receptor. In addition, it contributes to unconsciousness and amnesia via minor actions at the GABAa receptor. As seen in the EEG, ketamine is an excitatory agent and has been reported to increase cortical SSEP amplitude [70, 140] and increase the amplitude of myogenic and spinal recorded MEP responses. Ketamine also increases the H-reflex, suggesting a change in α motor neuron excitability may contribute to the MEP enhancement [82, 97, 101, 117, 141–145].
High dosages, however, produce depression of the myogenic response consistent with its known property of spinal axonal conduction block [146]. As such, ketamine can be added to an intravenous technique to enhance the antinociceptive effect while allowing reduction of agents, which produce depression of the evoked responses (e.g., propofol). Unfortunately, increases in intracranial pressure are seen in patients with cortical abnormalities. Hallucinatory activity can also be seen and is minimized by using midazolam and avoiding its use prior to awakening. Of note, ketamine is also thought to minimize acute opioid tolerance through its action at the NMDA receptor [147].
Lidocaine, Magnesium, and Regional Anesthesia
Lidocaine , when infused intravenously at a low dose, has been shown to reduce propofol and opioid doses in TIVA with maintenance of the cortical SSEP and myogenic MEP [148]. It is believed to act via GABA, NMDA, and sodium channels leading to contributions in unconsciousness, immobility, and antinociception. It has been used in patients who are opioid tolerant and is associated with reductions in postoperative pain. Similarly, magnesium infusion offers similar benefits but has not been fully explored with IOM [149]. It is thought to act similarly to ketamine through the NMDA receptor.
When used for regional anesthesia , local anesthetics block conduction in the neural pathways affected, causing loss of sensory and motor responses. This has been shown with epidural anesthesia [150–152], intravenous regional block [153], specific nerve blocks [154], thoracic paravertebral blocks [155], and topical anesthesia where stimulation is being conducted [156].
Neuromuscular Blocking Agents
Despite the controversy associated with the use of NMBA during IOM, a substantial amount of experience has been published. There are certain portions of surgical procedures where neuromuscular blockade (NMB) is routinely requested; in addition to intubation, NMB is often used during transabdominal approach to the anterior lumbar spine, during the initial portions of a posterior thoracic spinal procedure to reduce paraspinous muscle activity, and in interventional suites to facilitate a radiographic background “mask” to be subtracted. In addition, NMB is occasionally requested to prevent patient movement during microscopic surgery [157]. It should be noted that other techniques beside NMB have been used to reduce movement.
Some IOM techniques do not rely on muscle responses such that NMB can be used if they are the only responses measured. These include SSEP, VEP, or ABR [158]. To the extent they reduce electromyographic (EMG) noise in the recording, they may improve the signal-to-noise ratio and improve recording [158, 159].
Motor-Evoked Potentials
Because the myogenic MEP response and H-reflex is dependent on neural transmission through the neuromuscular junction (NMJ) , NMB will reduce the amplitude of the response and complete NMB will prevent monitoring of the myogenic MEP [160, 161]. However, monitoring with the MEP in the spinal canal (D wave) will be possible and relaxation may improve recording because of reduction of the paraspinous muscle artifact from stimulation [29, 131].
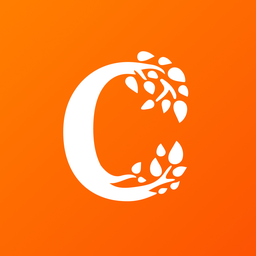
Full access? Get Clinical Tree
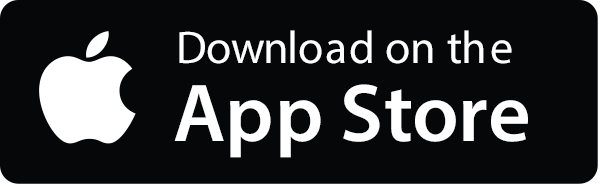
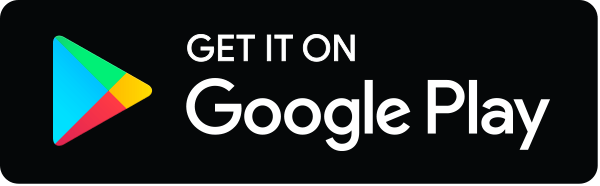