CHAPTER 22 Anesthesia for Neurosurgery
Developmental considerations
Intracranial Pressure
Normal intracranial pressure (ICP) is relatively low in premature infants and slightly higher in full-term infants (2 to 6 mm Hg) and children and adults (0 to 15 mm Hg). Intracranial compliance is defined as change in intracranial pressure relative to the intracranial volume (Fig. 22-1). At normal intracranial volumes (point 1 in Fig. 22-1), ICP is low but compliance is high and remains so despite small increases in volume. As intracranial volume acutely rises, the ability to compensate is rapidly overwhelmed. This occurs even when the ICP is still within normal limits, but the compliance is low (point 2 in Fig. 22-1). When ICP is already high, a threshold is reached, after which further volume expansion leads to rapid ICP elevation (point 3 in Fig. 22-1). Point 4 (see Fig. 22-1) reflects maximal intracranial volume and high ICP. Clinically, intracranial compliance can be assessed by a variety of devices that measure intracranial pressure (Wiegand and Richards, 2007).
The neonatal cranial vault is unique in that it is in a state of flux. Open fontanelles and cranial sutures lead to a compliant intracranial space (Fig. 22-2). The mass effect of a slow-growing tumor or insidious hemorrhage is often masked by a compensatory increase in intracranial volume accompanied by head growth. When ICP increases occur slowly, open fontanelles and cranial sutures separate and enlarge the intracranial space, and thus the mass effect of a slow-growing tumor or insidious intracranial bleeding is often shrouded by a compensatory increase in intracranial volume (widening of the fontanelles and cranial sutures). However, acute increases in cranial volume caused by massive hemorrhage or obstructed cerebrospinal fluid (CSF) flow cannot be attenuated by expansion of the cranial vault and frequently result in life-threatening intracranial hypertension in infants (Shapiro et al., 1980). Once the fontanelles and sutures have closed, children have a smaller cranial volume and lower intracranial compliance than adults. The posterior fontanelle is usually closed by 2 months of age. The anterior fontanelle closes after the posterior fontanelle. By 3 months of age, the anterior fontanelle is closed in 1% of infants. By 1 year it is closed in about 40%, and by 2 years it is closed in 96%. Contributory factors include a higher ratio of brain water content, less CSF volume, and a higher ratio of brain content to intracranial capacity (Arieff et al., 1992). Thus, children are at higher risk of herniation than adults.
Cerebral Blood Flow
The cerebral circulation is tightly regulated by a number of homeostatic mechanisms. The major influences of the cerebral circulation are metabolism, partial pressure of arterial carbon dioxide (Paco2), partial pressure of oxygen in arterial blood (Pao2), blood viscosity, and cerebral autoregulation. Coupling of flow and metabolism is the most significant regulator of the cerebral circulation and is preserved even during sleep (Madsen and Vorstrup, 1991; Lenzi et al., 2000) and during general anesthesia (Lam et al., 1995). Under normal conditions, cerebral blood flow (CBF) is tightly coupled to cerebral metabolism and cerebral metabolic rate of oxygen consumption (CMRo2) at global as well as regional levels. However, during periods of CNS activation, CBF increases more than CMRo2, resulting in a decrease in the cerebral oxygen extraction fraction (Fox and Raichle, 1986)
During development, CBF changes with age, mirroring changes in neural development. CBF increases during early childhood, peaking during early-to-mid childhood and plateauing at 7 to 8 years (Wintermark et al., 2004). The healthy brain receives about 15% of cardiac output, and normal adult CBF is approximately 50 mL/100 g per minute (Vavilala et al., 2002a). There are few data available from healthy children. Kennedy and Sokoloff (1957) found CBF to be much higher, in the order of 100 mL/100 g per minute, in conscious healthy children, and a study using arterial spin labeling found similar values in young children (Biagi et al., 2007), which then decreased and approached adult values during the teenage years. Even during low-dosage sevoflurane anesthesia, values for CBF in children are higher than those for adults, whose CBF ranges between 50 and 100 mL/100 g per minute (Settergren et al., 1980).
Transcranial Doppler (TCD) ultrasonography can be used to estimate CBF, assuming that changes in CBF are proportional to changes in cerebral blood flow velocity (CBFV), and that mean flow velocities are used to represent mean CBF. Systolic and diastolic flow velocity parameters are often used to examine resistance of the cerebral vessels and also brain death. Studies using TCD show that in healthy newborns, CBFV is approximately 24 cm/sec, thereafter increasing with age until 6 to 9 years (97 cm/sec) (Bode and Wais, 1988) (Fig. 22-3). Beyond 10 years of age, CBFV decreases, approximating adult values of about 50 cm/sec (Aaslid et al., 1984; Vavilala et al., 2002a). Sex differences have also been observed and may result from differences in hematocrit, hormones, vessel size, or cerebral metabolism (Kennedy and Sokoloff, 1957; Tontisirin et al., 2007). The mean CBFV estimates for middle cerebral artery (VMCA; anterior circulation) and basilar artery (VBAS; posterior circulation) by age and sex are listed in Figure 22-3 and Table 22-1 (Bode and Wais, 1988; Wintermark et al., 2004; Vavilala et al., 2005).
Cerebral Metabolic Rate
Global cerebral metabolic rate (CMR) for oxygen and glucose is higher in children than in adults (oxygen, 5.8 versus 3.5 mL/100 g brain tissue per minute, and glucose, 6.8 versus 5.5 mL/100 g brain tissue per minute, respectively) (Kennedy and Sokoloff, 1957). Like CMRo2, cerebral metabolic rate for glucose (CMRglu) is lower at birth (13 to 25 µmol/100 g per minute), increases during childhood, peaks by 3 to 4 years (49 to 65 µmol/100 g per minute), and remains high until 9 years of age. CMRglu decreases thereafter, approaching adult rates (19 to 33 µmol/100 g per minute) (Chugani et al., 1987); changes in CMRo2 and CRMglu mirror age-related changes in CBF (see Fig. 22-3).
Adenosine and nitric oxide (NO) are two purported mediators of flow–metabolism coupling and CBF increase with neuronal activation. Although the effect of adenosine on human CBF is controversial (Stange et al., 1997; Sollevi et al., 1987), up-regulation of NO production has been reported to increase CBF (Endres et al., 2004). Antagonists of both adenosine and NO attenuate the rise in CBF associated with neuronal activation, although neither mediator antagonist, alone or in combination, completely abolishes the CBF increase in response to neuronal activation (Gotoh et al., 2001). Therefore, other mediators, such as H+ ions, adenine nucleotides, potassium, prostaglandins, and vasoactive intestinal peptide, may also be involved in flow–metabolism coupling.
Hypothermia causes a reduction in CMRo2, thereby decreasing CBF via flow–metabolism coupling. CBF decreases approximately 5% to 7% per degree Celsius, and reduction of the brain temperature to 15° C will reduce CMRo2 to 10% of normothermic values (Vavilala et al., 2002). Hypothermia causes a reduction in both the basal metabolism required for maintenance of cellular integrity and the functional metabolism of the CNS.
Carbon Dioxide Vasoreactivity
Cerebral circulation is exquisitely sensitive to changes in Paco2 (Harper and Glass, 1965; Padayachee et al., 1986). Like that of CBF and metabolism, the vasoreactivity of CO2 may be higher in healthy children than in adults (13.8% and 10.3% change in mean CBFV per 1 mm Hg change in end-tidal CO2) during propofol anesthesia (Leon and Bissonnette, 1991; Rowney et al., 2002; Karsli et al., 2003) The cerebrovascular response to changing CO2 is attenuated at low blood pressure (Harper and Glass, 1965), increases in the presence of moderate hypoxia, decreases again during severe hypoxia (Quint et al., 1980), and may remain abnormally low after a hypoxic episode. Although there is an overall paucity of information regarding CO2 reactivity in healthy children and in pediatric disease states, older studies suggest that reactivity to CO2 is well developed even in healthy preterm infants (Pryds et al., 1990) and that CO2 reactivity in newborns correlates with the lowest pH and may reflect the severity of perinatal asphyxia (Baenziger et al., 1999).
There are many potential control mechanisms involved in reactivity to carbon dioxide (CO2R). Although neural regulation of CO2R may be important (Meyer et al., 1971; Bates et al., 1977; Rovere et al., 1977), the most prominent proposed mechanism is the biochemical/metabolic control of cerebral vasoreactivity. The brisk response of the cerebral vasculature to CO2 (the biochemical mechanism) is caused by the rapid diffusion of arterial CO2 across the blood-brain barrier (BBB) into the perivascular fluid and cerebral vascular smooth muscle. Changes in extracellular fluid pH by both CO2 and bicarbonate ions lead to cerebral vasodilation and increased CBF (Kontos et al., 1977). Although CO2 is a potent cerebral vasodilator, the role of arterial H+ ions is more controversial. Although pH is not known to generally affect the cerebral vasculature, because arterial H+ ions do not readily diffuse across the intact BBB (Harper and Bell, 1963), severe metabolic acidosis may cause vasodilation and increase CBF (Westerlind et al., 1994). Therefore, severe metabolic acidosis and alkalosis may affect cerebral vascular tone, as do respiratory acidosis and alkalosis.
During chronic hypercapnia maintained for 6 hours, an adaptive increase in the pH of the CSF occurs with a decrease in CBF (Warner et al., 1987). A decrease in pH is accompanied by an increase in CSF bicarbonate. Similarly, during chronic hypocapnia, CSF bicarbonate concentration decreases, CSF pH gradually decreases, and CBF increases (Muizelaar et al., 1988).
The mechanism of CO2R also appears to be regulated by local mediators rather than by chemoreceptors in the periphery. NO is partially responsible for CO2-mediated cerebral vasodilation. Schmetterer and coworkers (1997) demonstrated a significant reduction in mean VMCA to hypercapnia in healthy humans after administration of an NO synthase (NOS) inhibitor. However, NOS inhibitors do not completely ablate CO2R, and NO may be more important in regional than in global regulation of vasoreactivity. The cerebral cortex in primates was the only site in which NOS inhibitor attenuated the CBF response to increasing arterial CO2 concentration (McPherson et al., 1995). Another putative mediator of CO2R is prostaglandin (PG) E2. In contrast, indomethacin (a cyclooxygenase inhibitor) was shown to inhibit prostanoid synthesis and completely abolished CO2-induced cerebral vasodilation (Wagerle and Degiulio, 1994).
Hypoxic and Hyperoxic Control of CBF
Compared with Paco2, the influence of Pao2 on the cerebral circulation is of much less clinical significance. There are minimal changes in CBF with changes in Pao2 to greater than 50 mm Hg. Below that threshold of 50 mm Hg, CBF increases to maintain adequate cerebral oxygen delivery. Unlike CO2R, the equilibration of CBF is longer and takes approximately 6 minutes after the establishment of hypoxemia (Ellingsen et al., 1987).
Hypoxemia may induce cerebral vasodilation via anaerobic glycolysis and lactic acid production, causing decreased extracellular pH and subsequent vasodilation. However, pH changes during hypoxemia are only partially responsible for the increased CBF (Koehler and Traystman, 1982). Adenosine has been shown to be necessary for the vasodilatory response to hypoxemia (Morii et al., 1987; Brian et al., 1996; DiGeronimo et al., 1998). NO has also been implicated as a mediator, because NOS inhibitors attenuate the increase in CBF that occurs during hypoxemia (Hudetz et al., 1998). Opioids such as methionine enkephalin and leucine enkephalin have been observed to contribute to hypoxic pial artery dilation in the piglet. Vasopressin-induced CSF methionine enkephalin and leucine enkephalin release are attenuated in the presence of cyclic adenosine monophosphate (cAMP) and cyclic guanosine monophosphate (cGMP) antagonists. These data show that both cAMP and cGMP contribute to vasopressin-induced pial artery dilation and the release of the opioids methionine enkephalin and leucine enkephalin (Rossberg and Armstead, 1997).
Although hypoxemia produces cerebrovasodilation, the influence of increases in Pao2 (hyperoxia) at normal atmospheric pressure is less well characterized and somewhat controversial (Armstead, 1998). Previous reports have documented both decrease in CBF (Kennedy et al., 1972) and no change in CBF (Busija et al., 1980) during hyperoxia. Animal studies demonstrate that hyperoxia elicits pial artery vasoconstriction during normocapnia, and that vasoconstrictor peptide endothelin-1 (ET-1) contributes to that vascular response (Armstead, 1999).
Effects of Viscosity on CBF
Viscosity of blood is primarily a function of hematocrit (Hct), and decrease in viscosity is usually secondary to hemodilution. During anemia, CBF increases as a result of improved rheology of the blood flow in the cerebral vessels and as a compensatory response to decreased oxygen delivery (Tomiyama et al., 1999). Although some data suggest that an Hct of less than 30% is associated with worse Glasgow Outcome Scale scores at discharge for adults with severe traumatic brain injury (TBI) (Carlson et al., 2006), neither the optimal duration for maintaining a specific hemoglobin (Hgb) level nor the relationship between target transfusion and neurologic outcome is fully known (Timmons, 2006). A recent study of critically ill children demonstrated that maintaining a Hgb of 7 g/dL rather than 9.5 g/dL can reduce requirements for blood transfusion, but none of these subjects had TBI (Lacroix et al., 2007).
Cerebral Autoregulation
Cerebral autoregulation is a homeostatic process: arterioles dilate and constrict to maintain CBF nearly constant over a range of blood pressures. In healthy adults, changes in mean arterial pressure (MAP) between 60 and 160 mm Hg result in little or no change in CBF (Fig. 22-4) (Lassen, 1959; Paulson et al., 1990). This adaptive mechanism maintains constant (adequate) CBF by vasodilation or decreasing cerebrovascular resistance. Beyond these limits of autoregulation, hypotension may result in cerebral ischemia, and hypertension may cause cerebral hyperemia.
Healthy infants appear to autoregulate CBF as well as older children during low-dosage sevoflurane anesthesia, but the long-held assumption that the lower limit of autoregulation (LLA) is lower in young than in older children may not be valid (same LLA range for younger and older children, 46 to 76 mm Hg) (Vavilala et al., 2003). There are no data on the LLA in healthy neonates. Because blood pressure increases with age, young children may be at increased risk of cerebral ischemia as a result of lower blood pressure reserve (mean arterial pressure to LLA); however, neonates are especially vulnerable to cerebral ischemia and intraventricular hemorrhage because of this narrow autoregulatory range (Pryds et al., 2005) (Fig. 22-5). Sick premature neonates have CBF pressure-passivity, resulting in a linear correlation between CBF and systemic blood pressure (Boylan et al., 2000). Therefore, tight blood pressure control is essential in the management of neonates to minimize both cerebral ischemia during hypotension and intraventricular hemorrhage with increased blood pressure. Techniques such as deliberate hypotension in very young children or infants may not be desirable. Age-related increased latency may also occur in cerebral autoregulatory response in young children (Vavilala et al., 2002a), but no data exist in neonates. Animal data suggest that although CBF pressure autoregulation and reactivity to CO2 operate in the newborn rat, hypercapnia abolishes cerebral autoregulation (Pryds et al., 2005), that abolished autoregulation is associated with cerebral damage in asphyxiated infants, and that the combination of isoelectric electroencephalograms (EEGs) and cerebral hyperperfusion is an early indicator of very severe brain damage (Pryds et al., 1990).
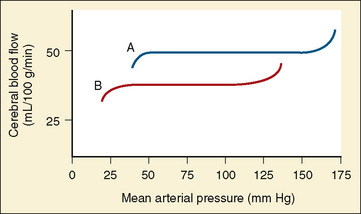
FIGURE 22-5 Autoregulation of cerebral circulation in neonates (curve B) and adults (curve A).
(Redrawn from Harris MM: Pediatric neuroanesthesia. In Berry FA, editor: Anesthetic management of difficult and routine pediatric patients, ed 2, New York, 1990, Churchill Livingstone, p 341. After data of Hernández MJ, Brennan RW, Vannucci RC, et al: Cerebral blood flow and oxygen consumption in the newborn dog, Am J Physiol 234:R209, 1978.)
Despite these clinical observations, the mechanisms of normal cerebral autoregulation in healthy children and adaptations in acute disease are not completely understood, and as occurs with changes in CBF, both anatomic and physiologic maturation might play a role in the development of a fully developed autoregulatory response. The mechanisms of cerebral autoregulation may involve a combination of myogenic, neurogenic, and metabolic processes. The metabolic mechanism stipulates that autoregulation is mediated by the release of a vasodilator substance that regulates the cerebrovascular resistance to maintain CBF constant. Although no specific substance fits all experimental observations, adenosine, a potent cerebral vasodilator, is formed from the breakdown of ATP when neuronal demand of oxygen exceeds supply (Winn et al., 1985). Adenosine can be found in increased concentration in cerebral tissue as systemic blood pressure falls toward the lower limit of autoregulation. In fact, brain adenosine concentration doubles within 5 seconds of decreasing blood pressure (Winn et al., 1980). Cortical activation via contralateral peripheral stimulation is also immediately followed by adenosine release and regional vasodilation (Ngai et al., 1998). It has been suggested that NO exerts an influence on basal and stimuli mediated cerebrovascular tone. The mechanism of NO-induced cerebral vasodilation probably involves cGMP and a decrease in intracellular calcium. It is unclear to what extent NO affects cerebral autoregulation in both healthy patients and in patients with TBI. Although earlier studies suggest that NO has no influence on cerebral autoregulation, Jones and coworkers (1999) described an increase in the lower limit of autoregulation with NOS inhibitors. Other transmitters or substances that have been proposed as mediators of autoregulation include protein kinase C (Jones et al., 1999), melatonin (Régrigny et al., 1999), prostacyclin, activated potassium channels, and intracellular second messengers (Faraci and Heistad, 1998).
The Neurogenic Mechanism
Perivascular innervation of the cerebral resistance vessels and the specific neurotransmitter contained in the perivascular nerve fibers may also modulate vascular response to change in blood pressure. However, the specific mechanisms by which the CNS exerts control on the cerebral vasculature are poorly understood. Although acetylcholine is the most abundant perivascular neurotransmitter, the list of neurotransmitters involved in this neural response includes norepinephrine, neuropeptide Y, cholecystokinin, acetylcholine, vasoactive intestinal peptide, and calcitonin gene-related peptide (Yoon et al., 1997). Experimentally sympathetic stimulation can shift the autoregulatory curve to the right, thus protecting the brain against severe elevation of MAP.
Effects of drugs on neurophysiology
The effects of the commonly used anesthetic agents on CBF, CMRo2, and CSF dynamics are discussed here and summarized in Table 22-2. The data are scant on these effects in infants and children. The responses in children are assumed to be the same as those in adults.
TABLE 22-2 Anesthetic Agents and Their Effects on Cerebral Hemodynamics and Neurophysiologic Monitors
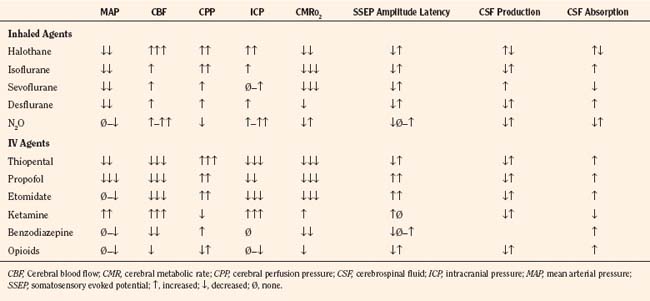
Intravenous anesthetic drugs have varied effects on cerebral hemodynamics. Thiopental and propofol maintain autoregulation and coupling and potently reduce CBF, cerebral blood volume (CBV), and CMRo2. Karsli and associates (2004) found that propofol maintained cerebrovascular reactivity to CO2 at levels greater than 30 mm Hg end-tidal CO2 in children. Furthermore, propofol, at high dosages, lowers CBFV and MAP values in children, which may be mediated by its cerebral vasoconstrictive properties (Karsli et al., 2002). Both thiopental and propofol have been touted as having neuroprotective properties, especially in preclinical studies, but their clinical efficacies in neurologically compromised pediatric patients have not been tested. Etomidate and ketamine are frequently used to induce anesthesia in hemodynamically compromised patients, as these drugs are less likely to cause hypotension than thiopental or propofol. However, CNS excitation and increased ICP have been associated with these drugs, respectively, and they may not be appropriate for many neurosurgical patients.
Intravenous Anesthetics
Propofol
Propofol is an intravenous (IV) induction agent with cerebrovascular properties similar to those of thiopental: both may depress systemic blood pressure but both potently decrease CMRo2, CBF, and ICP (Van Hemelrijck et al., 1990). Cerebral autoregulation and cerebral responsiveness to changes in arterial CO2 tension (Karsli et al., 2004) are well preserved during propofol anesthesia. Propofol is a good alternative to thiopental for induction of anesthesia for neurosurgery. In the presence of nitrous oxide and hypocapnia, propofol results in lower ICP, higher cerebral perfusion pressure (CPP), and less cerebral cortical edema than isoflurane or sevoflurane. In patients with or at risk for intracranial hypertension and decreased cerebral perfusion, maintenance of anesthesia with propofol is superior to inhaled halogenated anesthetics, at least until the dura has been opened.
Barbiturates
Barbiturates decrease CBF, CBV, and CMRo2 in a dosage-dependent manner (Pierce et al., 1962; Michenfelder, 1974; Albrecht et al., 1977) and therefore reduce ICP. Neither CBF nor cerebral metabolism is significantly altered by subanesthetic dosages of barbiturates. When the EEG becomes isoelectric, CBF and CMRo2 decrease to about 50% of normal, and additional doses of barbiturates have little further effect. Barbiturates may also be used to prevent increases in ICP that can occur with laryngoscopy and endotracheal intubation. Autoregulation and the cerebrovascular response to changes in Paco2 remain intact during barbiturate anesthesia. The rate of CSF formation and the resistance to reabsorption of CSF are not altered by barbiturates (Mann et al., 1979). In dosages that suppress the EEG, barbiturates reduce cerebral damage in animal and human models of focal cerebral ischemia (Smith et al., 1974; Nehls et al., 1987). In animals, barbiturates also reduce the extent of cerebral edema after a cortical freeze injury. This decrease in edema is in contrast to the response observed with the volatile anesthetics (Smith and Marque 1976).
Opioids
Opioids have little effect on CBF, CBV, or ICP unless respiration is depressed and Paco2 is increased (Miller et al., 1975; Misfeldt et al., 1976; Jobes, 1977; Moss et al., 1978).
Fentanyl
When combined with nitrous oxide, fentanyl decreases CBF by 47% and CMRo2 by 18% (Michenfelder, 1974). Autoregulation and CO2 responsiveness of the cerebral circulation are not altered. Fentanyl does not alter the rate of CSF formation, but it reduces the resistance to CSF reabsorption by 50% (Artru, 1984a, 1984c), the effect of which is to decrease CSF volume to a degree of unknown clinical significance. The neonatal cerebral circulation is also unaffected by fentanyl (Yaster et al., 1987). Sufentanil and alfentanil in high dosages (10 to 20 mcg/kg) reduce both CBF and CMRo2 by 25% to 30% (Stephan et al., 1991), whereas at exceedingly high dosages (10 to 200 mcg/kg), sufentanil may transiently increase CBF while reducing CMRo2 (Milde et al., 1990). However, at conventional dosages, sufentanil (Herrick et al., 1991; Weinstabl et al., 1991; Mayberg et al., 1993) does not appear to have adverse effects on the cerebral vasculature or on ICP in most patients. In a subset of patients with severe head injuries and very poor intracranial compliance, sufentanil may cause a small (e.g., <10 mm Hg) and transient increase in ICP that may be clinically significant in some settings (Sperry et al., 1992; Weinstabl et al., 1992; Albanese et al., 1993).
Remifentanil
Remifentanil is an ultra-short-acting to short-acting opioid that is rapidly metabolized by plasma and tissue esterases. The very short clinical duration of effect of remifentanil and its context-sensitive half-life, which is independent of the duration of infusion (Minto et al., 1997; Mertens et al., 2003), make it an appealing opioid for lengthy neurosurgical procedures after which rapid return of consciousness is desirable. As is the case with other opioids that have been studied, remifentanil does not increase CBF or ICP (Hoffman et al., 1993; Baker et al., 1997; Ostapkovich et al., 1998; Klimscha et al., 2003; Engelhard et al., 2004; Lagace et al., 2004). Return of consciousness is very rapid after remifentanil is discontinued, and the frequency of administration of naloxone to permit neurologic assessment is decreased (Guy et al., 1997). However, because remifentanil analgesia is very brief after its discontinuation, a long-acting opioid analgesic must be administered to prevent severe pain and rebound hypertension before or soon after remifentanil is discontinued (Gerlach et al., 2003; Cafiero et al., 2004).
Other Intravenous Anesthetics
Etomidate reduces ICP by decreasing CBF and CMRo2 by 34% and 45%, respectively. It, too, preserves the CO2 responsiveness of the cerebral circulation (Renou et al., 1978; Moss et al., 1979). A side effect of etomidate administration is myoclonus, which has been reported after prolonged continuous infusion of etomidate (Laughlin and Newberg, 1985). Lidocaine in clinical dosages decreases CBF and reduces the increase in ICP associated with endotracheal intubation (Sakabe et al., 1974; Donegan and Bedford, 1980). The benzodiazepines (diazepam, lorazepam, and midazolam) decrease CBF and CMRo2 by approximately 25% (Cotev and Shalit, 1975; Rockoff et al., 1980; Tateishi et al., 1981; Forster et al., 1982; Nugent et al., 1982; Nakahashi et al., 1991).
Dexmedetomidine
The neurophysiologic effects of dexmedetomidine (an α2-agonist) have not been extensively studied in humans. In animals, it has been noted to decrease CBF but was not associated with a proportional decrease in the cerebral metabolic rate (Zornow et al., 1990). In human studies, CBFV decreases after dexmedetomidine administration (Zornow et al., 1993) and CBF—both global and regional—decreases by 30% at clinically relevant concentrations of dexmedetomidine (Prielipp et al., 2002).
The effect of dexmedetomidine on ICP in patients with space-occupying lesions is unclear. In patients undergoing transsphenoidal hypophysectomy, dexmedetomidine had no effect on lumbar CSF pressure (Talke et al., 1997). Its effect on the amplitude and latency of cortical somatosensory evoked potential is minimal.
Ketamine
In contrast to the other IV anesthetic agents, ketamine is a potent cerebrovasodilator. It increases CBF by 60% with little change in CMRo2 (Dawson et al., 1971; Takeshita et al., 1972; Schwedler et al., 1982). The cerebrovascular response to administration of ketamine is thought to be the result of regional cerebral activation induced by the drug (Hougaard et al., 1974). Ketamine produces a marked increase in ICP, which can be reduced, but not prevented, by hyperventilation (Gardner et al., 1972; Takeshita et al., 1972; Wyte et al., 1972). The increase in CBF, and presumably in ICP, can be blocked by previous administration of thiopental (Dawson et al., 1971). Ketamine has been associated with sudden elevation of ICP and clinical deterioration when used in patients with hydrocephalus and other intracranial pathology (List et al., 1972; Lockhart and Jenkins, 1972; Wyte et al., 1972). Although ketamine is not currently used as a general anesthetic in patients with reduced intracranial compliance, a recent study in critically ill children reported a decrease in ICP following ketamine (Bar-Joseph et al., 2009), thereby challenging the notion that ketamine increases ICP.
Inhalational Anesthetics
Volatile anesthetic drugs are potent cerebrovasodilators and may produce uncoupling of CMRo2 and CBF, and different volatile anesthetics may have varying uncoupling profiles (Hansen et al., 1989). Uncoupling may lead to increased CBV and can exacerbate intracranial hypertension. Children have increased sensitivity to the vasodilatory effects of volatile drugs. TCD measurements reveal that the following volatile agents increase CBFV in descending order: halothane, desflurane, isoflurane, and sevoflurane. Autoregulation is also blunted in the presence of volatile drugs in a dosage-dependent fashion. However, this perturbation is mitigated by hypocapnia. Nitrous oxide has vasodilatory effects alone and in combination with other anesthetics. Because isoflurane and sevoflurane significantly decrease CMRo2 in children and maintain coupling, these two inhaled drugs are ideal anesthetics for the neurologically compromised patient. Sevoflurane does not significantly affect CBFV, and it preserves cerebral autoregulation in children anesthetized with up to a minimum alveolar concentration (MAC) of 1.5 (Fairgrieve et al., 2003; Wong et al., 2006).
Nitrous Oxide
Nitrous oxide is a weak cerebrovasodilator whose effects on CBF are offset by hyperventilation and barbiturate anesthesia (Algotsson et al., 1988). The variability of effects that nitrous oxide has on CBF and ICP in different reports results from differences in experimental species and background anesthesia. In many animals, nitrous oxide in subanesthetic dosages (60% to 70%) causes excitement and cerebral metabolic stimulation, with an accompanying increase in CBF (Theye and Michenfelder, 1968; Sakabe et al., 1978; Pelligrino et al., 1984; Drummond et al., 1987). Because nitrous oxide is not an adequate anesthetic in the absence of other inhalation or IV anesthetics, the modification of its cerebral effects by additional anesthetic drugs is particularly important. Nitrous oxide at 70% does not, for example, cause a change in CBF, but it does reduce CMRo2 by 15% to 20% during barbiturate and narcotic anesthesia (Sakabe et al., 1978). However, when nitrous oxide is added to a volatile anesthetic such as isoflurane (Cucchiara et al., 1974; Manohar and Parks, 1984) or halothane (Sakabe et al., 1976), both CBF and CMRo2 increase. When nitrous oxide is added to sevoflurane, cerebral hyperemia increases and autoregulation is impaired (Iacopino et al., 2003).
The cerebrovascular responses to changes in Paco2 and MAP are preserved during nitrous oxide anesthesia. ICP may increase in response to nitrous oxide in patients with intracranial mass lesions and reduced intracranial compliance (Henriksen and Jörgensen, 1973; Moss and McDowall, 1979; Iacopino et al., 2003). The increase in ICP with nitrous oxide, however, is readily reversible by diazepam and barbiturate anesthesia and simultaneously initiated hyperventilation (Phirman and Shapiro, 1977).
The use of nitrous oxide for pediatric neuroanesthesia remains controversial. Some anesthesiologists prefer to avoid it because of its ability to increase CMRo2 and reduce the cerebroprotective effects of barbiturates. Others are concerned because it readily diffuses into collections of intracranial air and may increase ICP in the presence of pneumocephalus (Artru, 1982; Skahen et al., 1986). Asymptomatic accumulation of intracranial air occurs commonly during craniotomies, especially those associated with posterior fossa surgery and drainage of CSF (Yates et al., 1994). Some anesthesiologists discontinue nitrous oxide before closure of the dura to reduce the incidence of tension pneumocephalus, and others administer nitrous oxide throughout the procedure without any obvious detrimental effects. Indeed, one randomized control trial comparing anesthetic techniques with and without nitrous oxide in patients undergoing sitting craniotomies showed no difference in the incidence or size of pneumocephalus between the three groups (Hernández-Palazón et al., 2003). It may be that nitrous oxide equilibrates with intracranial air before the dura is closed; if so, ICP would not increase during craniodural closure because air pockets would already contain nitrous oxide. In addition, the discontinuance of nitrous oxide would decrease ICP, as nitrous oxide diffused back into the bloodstream (Skahen et al., 1986). Maintenance with nitrous oxide until the end of the surgery may be advantageous because it permits rapid awakening and may reduce the intracranial gas volume and the likelihood of delayed tension pneumocephalus. Nitrous oxide is generally not contraindicated during sitting craniotomies, even though the volume of a venous air embolus (VAE) expands in the presence of nitrous oxide. In fact, this phenomenon actually increases the sensitivity of monitoring for VAE by capnography, while at the same time nitrous oxide neither increases the risk of VAE (Losasso et al., 1992) nor increases the hemodynamic consequences of VAE, provided that nitrous oxide is discontinued when VAE is first detected (Losasso et al., 1992).
Isoflurane
Isoflurane is frequently used for neuroanesthesia. Its popularity is based on the fact that it affects CBF less than does halothane at equivalent MAC dosages (Todd and Drummond, 1984; Drummond and Todd, 1985; Algotsson et al., 1988), the fact that 1 MAC isoflurane preserves cerebral autoregulation (McPherson and Traystman, 1988) and CO2 responsiveness (McPherson et al., 1989), and the belief that it may provide cerebral protection (Newberg and Michenfelder, 1983; Verhaegen et al., 1992). Cerebral autoregulation is less affected by isoflurane than by halothane (Todd and Drummond, 1984b). In addition, isoflurane does not change CSF production, and it reduces the resistance to reabsorption of CSF (Artru, 1984b). During hypocapnia, CBF is lower with 1.0 MAC isoflurane (with 75% nitrous oxide) than with nitrous oxide alone (Cucchiara et al., 1974; Drummond and Todd, 1985). In contrast, 1.0 MAC halothane (with 75% nitrous oxide) increases CBF. Despite their dissimilar effects on CBF, isoflurane and halothane increase ICP equally in an animal model of brain injury (Scheller et al., 1987). This is probably because isoflurane and halothane increase CBV to a similar degree (Artru 1984c; Archer et al., 1987). In patients with reduced intracranial compliance, isoflurane increases ICP. This increase can be attenuated by simultaneous initiation of hyperventilation (Adams et al., 1981).
Isoflurane may be safely used in patients with small supratentorial brain tumors (Madsen et al., 1987), but it may cause dangerous increases in ICP in patients with large intracranial mass lesions that are associated with a midline shift evident on a computed tomography (CT) scan (Grosslight et al., 1985). Like halothane, isoflurane should be avoided in patients with reduced intracranial compliance until the dura is open, if ICP is not being monitored. Isoflurane decreases CMRo2 by 30%, and it causes an isoelectric EEG at concentrations above 2.0 MAC (Newberg and Michenfelder, 1983). It is unique among the volatile agents in that it preserves normal cerebral energy states and aerobic metabolism at very low blood pressure (40 mm Hg), in contrast to the findings observed with hypotension induced by halothane, trimethaphan, or sodium nitroprusside (Newberg et al., 1984). In studies of mice exposed to 5% oxygen, isoflurane increased survival time and thus may have provided some degree of cerebral protection. In studies of incomplete global ischemia in isoflurane-anesthetized dogs, cerebral energy stores were increased, presumably through depression of cortical electrical activity and cerebral metabolism (Newberg and Michenfelder, 1983). Protective effects of isoflurane, however, were not observed in a primate model of regional cerebral ischemia (Nehls et al., 1987).
Sevoflurane
Sevoflurane is a fluorinated ether with a low blood-gas solubility. Studies in rabbits suggest that sevoflurane does not increase CBF at 0.5 to 1.0 MAC. Sevoflurane does cause increases in CBF and ICP and a decrease in cerebral oxygen consumption (Scheller et al., 1988) and CPP, similar to isoflurane (Petersen et al., 2003). Compared with isoflurane, sevoflurane allows more rapid emergence after lengthy neurosurgery, and thus more rapid neurologic assessment (Mönkhoff et al., 2001; Gauthier et al., 2002). Taken together, the available evidence suggests that sevoflurane is a more appropriate inhaled anesthetic than halothane during craniotomy, and that it is equivalent in its cerebrovascular effects to isoflurane, while allowing more rapid recovery after long anesthesia. As is the case with other halogenated agents, if ICP or intracranial compliance is compromised, sevoflurane should be withheld until the dura has been opened (Petersen et al., 2003).
Halothane
Halothane is a cerebral vasodilator that decreases cerebrovascular resistance and increases CBF in a dosage-dependent fashion (Wollman et al., 1964; Albrecht et al., 1977; Todd and Drummond, 1984; Brüssel et al., 1991). The increase in CBF is transient; CBF decreases to baseline levels after 150 minutes of halothane anesthesia (Albrecht et al., 1983). CBV, however, remains elevated by 11% to 12% over a 3-hour period of halothane administration (Artrub, 1983). Halothane reduces CMRo2 by 17% to 33% (Albrecht et al., 1977). The cerebral vasculature remains responsive to changes in arterial Paco2 (Alexander et al., 1964; Wollman et al., 1964; Drummond and Todd, 1985). Halothane in high concentrations (2.0 MAC) abolishes autoregulation of the cerebral circulation in response to changes in MAP in both adults (Miletich et al., 1976; Todd and Drummond, 1984) and infants (Messer et al., 1989). Halothane alters blood-brain barrier permeability, promoting the extravasation of plasma proteins into normal brain during periods of acute hypertension (Forster et al., 1978). Halothane reduces CSF formation by 30% in dogs and increases the resistance of reabsorption of CSF (Artru, 1983a, 1984b).
Because ICP is determined by CBV, CSF volume, and brain tissue volume, it is not surprising that ICP increases with halothane (Jennett et al., 1969; DiGiovanni et al., 1974). Peak increases are observed in 3 to 13 minutes, although the increase persists over 3 hours of halothane exposure (Artru, 1983b). The increase in ICP in patients with intracranial mass lesions can be attenuated, but not totally prevented, by establishing hyperventilation for 10 minutes before the introduction of halothane (Adams et al., 1981). If ICP is not being monitored, halothane should not be used in patients with reduced intracranial compliance until the dura is open and its effects on the brain can be seen.
Desflurane
Desflurane is an inhalation agent that is chemically similar to isoflurane. Its physicochemical properties are remarkable, with a blood-gas partition coefficient even lower than that of nitrous oxide, permitting rapid uptake and washout of the gas. The effects of desflurane on cerebral metabolism and hemodynamics are not as well studied as the effects of the other inhalation agents, but animal studies suggest that its effects are not unique in any way. Desflurane at clinical concentrations is a potent cerebral vasodilator, increasing ICP (Artru, 1994), increasing CBF by 50% at 1.5 MAC, and reducing autoregulation of CBF (Lutz and Milde, 1990). However, cerebrovascular responsiveness to hypocapnia is preserved during desflurane anesthesia in laboratory animals, protecting the animal from increases in ICP if hyperventilation occurs during the anesthesia (Lutz et al., 1991; Young, 1992). In patients with mass lesions, equivalent MAC dosages of desflurane and isoflurane are similar in terms of absolute CBF, the response to increasing dosages, and the preservation of CO2 reactivity (Ornstein et al., 1993; Fraga et al., 2003). Desflurane effects seen on the EEG are also similar to those of isoflurane. At increasing concentrations, the electroencephalographic frequency decreases and the amplitude increases. Burst suppression appears at about 1.24 MAC (Rampil et al., 1991).
Muscle Relaxants
Succinylcholine
Succinylcholine is very infrequently used in pediatric anesthesia because of its association with life-threatening hyperkalemia and cardiac arrest in children with undiagnosed myopathies. This has led to a black-box warning by the U.S. Food and Drug Administration, reserving its use in children for emergency intubation when securing the airway is necessary. Most often, the use of even high-dosage nondepolarizing neuromuscular blockers is appropriate for emergently securing the airway in children, but in some cases a very rapid and immediate pharmacologic paralysis is required, and succinylcholine remains the drug of choice in these circumstances. Life-threatening hyperkalemia has also been associated with administration of succinylcholine after many types of CNS disorders, including closed-head injury, even without motor deficits (Mazze et al., 1969; Thomas, 1969; Smith and Grenvik, 1970), cerebral hypoxia caused by near-drowning (Tong, 1987), subarachnoid hemorrhage (Iwatsuki et al., 1980), encephalitis (Cowgill et al., 1974), cerebrovascular accidents (Cooperman et al., 1970), and paraplegia (Cooperman, 1970; Tobey, 1970). The onset of the period of vulnerability is not well defined. It may begin as early as 24 to 48 hours after injury and may last up to 1 to 2 years after injury (Cooperman, 1970). Because the period of risk for succinylcholine-induced hyperkalemia after cerebral injury is undefined, succinylcholine should be avoided in these patients, except in the period immediately after injury. Succinylcholine can increase CBF and ICP in patients with reduced intracranial compliance (Cottrell et al., 1983; Minton et al., 1986; Stirt et al., 1987a; Thiagarajah et al., 1988), probably because of cerebral stimulation from succinylcholine-induced increases in afferent-muscle spindle activity (Lanier et al., 1986.). The increases in CBF and ICP can be blunted by deep general anesthesia or by previous paralyzing or “defasciculating” dosages of nondepolarizing muscle relaxants (Minton et al., 1986; Stirt et al., 1987a). In contrast, most nondepolarizing relaxants have little effect on CBV and ICP (Lanier et al., 1985; Minton et al., 1985; Stirt et al., 1987b; Rosa et al., 1991), unless associated with histamine release (d-tubocurarine, atracurium), which causes transient cerebrovasodilation and increased ICP (Tarkkanen et al., 1974). In dosages that do not release histamine, atracurium does not increase ICP, despite the accumulation of laudanosine, a major metabolic product of atracurium and a potential CNS arousal agent.
Nondepolarizing Muscle Relaxants
The presence of motor deficits or the administration of anticonvulsants may affect the dosage of nondepolarizing muscle relaxant necessary in neurosurgical patients. Hemiplegia from an upper motor neuron lesion (such as a stroke or a brain tumor) is associated with resistance to nondepolarizing relaxants on the paretic side (Graham, 1980; Moorthy and Hilgenberg, 1980; Shayevitz and Matteo, 1985). Excessive dosages of muscle relaxants may be given if the dosage is guided by a nerve stimulator monitoring a hemiplegic extremity. In contrast, an increased response to nondepolarizing muscle relaxants is observed in paretic-muscle lower motor neuron lesions (e.g., paraplegia and quadriplegia) (Brown et al., 1975). Acute administration of several anticonvulsants, including phenytoin, phenobarbital, trimethadione, and ethosuximide, enhances nondepolarizing neuromuscular blockade or delays its reversal (Gandhi et al., 1976; Spacek et al., 1999). Patients receiving chronic phenytoin or carbamazepine therapy are resistant to the effects of nondepolarizing relaxants, including pancuronium, (Roth and Ebrahim, 1987), metocurine (Ornstein et al., 1985), vecuronium (Ornstein et al., 1987; Alloul et al., 1996), and rocuronium (Spacek et al., 1999; Hernández-Palazón et al., 2001) but, interestingly, not mivacurium or atracurium (Ornstein et al., 1987; Spacek et al., 1996). The cause of phenytoin-induced resistance to nondepolarizing muscle relaxants and the reason for the lack of the same effect with mivacurium or atracurium are unclear. Finally, no data have yet been published describing the interactions of anticonvulsants (felbamate, gabapentin, levetiracetam, tiagabine, topiramate, sodium valproate, or valproic acid) with nondepolarizing neuromuscular blocking drugs (Fig. 22-6).
Vasodilators
The direct-acting vasodilators, including sodium nitroprusside, ATP, adenosine, nitroglycerin, diazoxide, and hydralazine, are cerebrovasodilators and may increase CBF and ICP (Stoyka and Schutz, 1975; Turner et al., 1977; Marsh et al., 1979; Cottrell et al., 1980; McDowall, 1985). The calcium channel blockers also raise CBF and ICP (Griffin et al., 1983; Mazzoni et al., 1985). These drugs should therefore be avoided in patients with reduced intracranial compliance, unless the dura is open or ICP is monitored. Indirect-acting antihypertensives, including trimethaphan (a ganglionic blocker), propranolol and esmolol (β-adrenergic blockers), and labetalol (a combined α- and β-blocker), do not increase CBF or ICP (Magness et al., 1973; Turner et al., 1977; McDowall, 1985). These agents are useful for the control of blood pressure in patients with elevated ICP. Trimethaphan may interfere with the neurologic examination by causing mydriasis, cycloplegia, or anisocoria. Sodium nitroprusside lowers the range of cerebral autoregulation. Brain-surface oxygen tension is greater (Maekawa et al., 1977) and metabolic disturbances in brain biochemistry (e.g., lactate, pyruvate, and phosphocreatine levels) are less during nitroprusside-induced hypotension than with trimethaphan- or hemorrhage-induced hypotension (Michenfelder and Theye, 1977). In addition, cortical blood flow and electrical activity are better preserved at lower MAP with sodium nitroprusside (Ishikawa and McDowall, 1981). Nitroprusside, however, induces more pronounced blood-brain barrier dysfunction than does trimethaphan (Ishikawa et al., 1983). Because CBF, brain oxygen tension, neuronal function, and brain metabolism are better maintained with sodium nitroprusside than with trimethaphan at a MAP of 50 mm Hg, sodium nitroprusside is the preferred agent for deliberate hypotension.
Central Nervous System Toxicity of Anesthetic Agents
Preclinical studies have demonstrated that prolonged exposure of fetal and neonatal laboratory animals to anesthetic drugs leads to accelerated neurodegeneration (Ikonomidou et al., 1999; Jevtovic-Todorovic et al., 2003; Slikker et al., 2007). The data generated in these reports have provoked a heated debate on its relevance to the practice of pediatric anesthesia (Anand and Soriano, 2004; Olney et al., 2004). There is no doubt that this phenomenon is valid in the experimental paradigms used in these laboratory investigations. The same model has also implicated dexamethasone and magnesium sulfate in accelerating neuroapoptosis in the development of rat brain (Noguchi et al., 2008; Dribben et al., 2009). However, a number of factors make the extrapolation of these findings to humans in the daily practice of clinical pediatric anesthesiology questionable. For example, these animal and in vitro studies have significant limitations in terms of agent dosage and duration of exposure (both absolute and in comparison with human studies) (Loepke and Soriano, 2008). Furthermore, no defined clinical markers or syndromes are associated with neonatal exposure to general anesthetics. Unlike general anesthetic agents, exposure to alcohol or anticonvulsant drugs during gestational development has clearly characterized clinical syndromes. Although histologic evidence of accelerated neurodegeneration has been verified by several investigational groups, discrepancies in neurocognitive outcomes exist (Loepke et al., 2009). Prospective clinical investigations are underway to verify these observations. Finally, the majority of neonatal and infant surgery is urgent or emergent, and anesthetic care is essential to proceed safely. It has been clearly demonstrated that inadequate anesthesia leads to poor postoperative outcomes in infants (Anand and Hickey, 1987; Anand and Soriano, 2004), and it is crucial to attenuate the stress response associated with surgical stimulation.
Several retrospective reports have positively linked exposure to general anesthesia before the age of 4 with an increased chance of developing learning deficits. Wilder and colleagues (2009) examined the extensive medical and school records of 5358 children in Rochester, Minnesota, and determined that there was an association between learning deficits in school and having more than two surgeries before age 4. A cross-sectional study done by Kalkman and colleagues (2009) in Utrecht, The Netherlands, surveyed a small number of parents of children who had had surgery at a young age and determined that there was a trend toward greater prevalence of learning deficits in children who had had early surgery. However, these reports need to be reconciled with a recent report demonstrating no causal relationship between anesthesia exposure and subsequent learning behavior in twin cohorts (Bartels et al., 2009). Clinicians should be aware of the rapid developments in the area of anesthetic-induced neurodegeneration and keep in mind the degree of necessity of infant surgical procedures and the more significant effects of the hypoxic and cardiovascular mechanisms of brain injury and death during anesthesia and surgery in these patients.
Preoperative evaluation and preparation
A proper and thorough evaluation and preparation of the pediatric patient for anesthesia and surgery are essential components of minimizing perioperative morbidity and mortality. Because of the urgent nature of many pediatric neurosurgical procedures, a thorough preoperative evaluation may be difficult. The perioperative management of pediatric patients undergoing neurosurgical procedures should be based on the developmental stage of the patient and is discussed in Chapter 8, Psychological Aspects, and Chapter 9, Preoperative Preparation. Given the systemic effects of general anesthesia and the physiologic stress of surgery, an organ system–based approach is optimal for anticipating potential physiologic derangements and coexisting disease states that may increase the risk of perioperative complications (Ferrari, 1999). Many potential perioperative problems can be preemptively addressed with such an approach. Because some children are either preverbal or do not fully understand their medical condition, their parents or primary caregivers should be interviewed carefully to obtain information regarding coexisting medical problems. A thorough review of the patient’s history can reveal conditions that may increase the risk of adverse reactions to anesthesia and perioperative morbidity and identify patients who need more extensive evaluation or whose medical condition needs to be optimized before surgery. There are also special perioperative concerns regarding children with neurologic abnormalities. However, certain aspects of this evaluation are of special relevance to neurosurgical patients. Specific concerns in these patients are listed in Table 22-3. Preoperative laboratory testing should be tailored to the proposed neurosurgical procedure.
TABLE 22-3 Common Perioperative Concerns for Infants and Children with Neurologic Lesions
Condition | Anesthetic Implications |
Denervation injuries | Hyperkalemia after succinylcholine Resistance to nondepolarizing muscle relaxants Abnormal response to nerve stimulation |
Chronic anticonvulsant therapy | Hepatic and hematologic abnormalities Increased metabolism of anesthetic agents |
Arteriovenous malformation | Potential congestive heart failure |
Neuromuscular disease | Malignant hyperthermia Respiratory failure Sudden cardiac death |
Chiari malformation | Apnea Aspiration pneumonia Stridor |
Hypothalamic and pituitary lesions | Diabetes insipidus or syndrome of inappropriate antidiuretic hormone Hypothyroidism or hyperthyroidism Adrenal insufficiency or adrenal excess |
The chronicity and severity of the patient’s neurologic condition vary greatly and should dictate the perioperative management. Special attention should be given to symptoms of allergy to latex products, because anaphylaxis has been reported in a number of children who have undergone multiple previous operations, especially patients with meningomyeloceles (Holzman, 1997). Severe dehydration and electrolyte abnormalities can be the result of protracted vomiting from intracranial hypertension. Patients with diabetes insipidus can develop hypovolemia as a result of polyuria. During the perioperative period, steroids are frequently initiated to palliate cerebral swelling in patients with intracranial tumors. Therapeutic levels of anticonvulsants should be verified preoperatively and maintained perioperatively. Patients on long-term anticonvulsants may develop toxicity, especially if seizures are difficult to control. This reaction frequently manifests with abnormalities in either hematologic or hepatic function, or both. Patients on chronic anticonvulsant therapy may also require increased amounts of sedatives, nondepolarizing muscle relaxants, and narcotics because of enhanced metabolism of these drugs (Soriano et al., 2000b; Soriano et al., 2001; Soriano and Martyn, 2004).
Physical Examination
The physical examination should include a brief neurologic evaluation that includes consciousness level, motor and sensory function, normal and pathologic reflexes, integrity of the cranial nerves, and signs and symptoms of intracranial hypertension (Fig. 22-7 and Table 22-4). It is essential to document these factors in the preoperative examination to be able to gauge postoperative neurologic function. Lesions of the brainstem can manifest with cranial nerve dysfunction such as respiratory distress, impaired gag reflex and swallowing, and pulmonary aspiration. Evidence of muscle atrophy and weakness should be noted, particularly if the patient is hemparetic, hemiplegic, or bedridden, because up-regulation of acetylcholine receptors may precipitate sudden hyperkalemia after succinylcholine administration and induce resistance to nondepolarizing muscle relaxants in the affected limbs. Body weight should be accurately measured to guide the administration of drugs, fluids, and blood products. Physical signs of dehydration should be noted, especially in patients who have been chronically ill or have received osmotic or diuretic agents.
TABLE 22-4 Signs of Intracranial Hypertension in Infants and Children
Infants | Children | Infants and Children |
Irritability | Headache | Decreased consciousness |
Full fontanelle | Diplopia | Cranial nerve (III and VI) palsies |
Widely separated cranial sutures | Papilledema | Loss of upward gaze (setting-sun sign) |
Cranial enlargement | Vomiting | Signs of herniation, Cushing’s triad, pupillary changes |
Radiologic and Laboratory Evaluation
Most neurosurgical patients have a magnetic resonance imaging (MRI) or CT scan of the head as part of the preoperative assessment. These scans should be reviewed with the neurosurgeon to confirm the primary lesion and the presence of evolving neurologic conditions (hydrocephalus, compressed cisterns, midline shifts) (Fig. 22-8). Preoperative laboratory tests should be tailored to the proposed neurosurgical procedure. Given the risk of significant blood loss associated with surgery, the hematocrit, prothrombin time, and partial thromboplastin time should be obtained to uncover any insidious hematologic disorder. Patients with suprasellar pathology should have an endocrinology evaluation. Type- and cross-matched blood should be ordered before all craniotomies. Endocrinologists may be needed to help optimize the patient’s condition before surgery and to assist in postoperative management. Liver function tests and a hematologic profile should be obtained if not recently reviewed for children taking long-term anticonvulsants.
Premedication
Perioperative anxiety plays a significant role in the care of the pediatric neurosurgical patient. Anxiety issues are related to the cognitive development and age of the child (see Chapter 2, Behavioral Development). Preoperative sedatives given before the induction of anesthesia can ease the transition from the preoperative holding area to the operating room (Kain et al., 2004). Oral midazolam is particularly effective in relieving anxiety and producing amnesia. If an indwelling IV catheter is in place, midazolam can be slowly titrated to achieve sedation. Sedation is usually withheld from pediatric neurosurgical patients until they arrive in the preoperative area to avoid the problem of an oversedated, neurologically impaired patient with inadequate supervision; however, heavy premedication may be warranted to avoid agitation in patients with moyamoya disease or an intracranial aneurysm or arteriovenous malformation that has recently bled. Narcotics are best withheld preoperatively, as they may cause nausea or respiratory depression, especially in patients with increased ICP.
General principles of intraoperative management
Induction of Anesthesia
The patient’s preoperative status dictates the appropriate technique and drugs for induction of anesthesia. A modified Glasgow Coma Scale for infants and children is useful for assessing the mental status of the patient before the administration of anesthesia (Table 22-5). Somnolent patients with intracranial hypertension are at risk for aspiration pneumonitis and require a rapid-sequence induction of anesthesia.
In the presence of intracranial hypertension, the primary goal during induction is to minimize severe increases in ICP. The effects of anesthetic drugs on cerebral hemodynamics were discussed extensively earlier in this chapter and are summarized in Table 22-2. In general, most IV drugs decrease CBF, metabolism, and ICP. Thiopental (4 to 8 mg/kg) and propofol (2 to 5 mg/kg) have similar effects on cerebral hemodynamics and maintain tight coupling of CBF and cerebral metabolic rate. Patients at risk for aspiration pneumonitis (including certain patients with intracranial hypertension) should have rapid-sequence induction of anesthesia using cricoid pressure. An IV hypnotic drug, such as thiopental or propofol, is administered and followed immediately by a rapid-acting muscle relaxant. Rocuronium can be used when succinylcholine is contraindicated, such as for patients with spinal cord injuries or paretic extremities. In instances of preexisting neurologic injury (e.g., in a patient with a history of a stroke resulting in a weak extremity), succinylcholine can result in sudden, catastrophic hyperkalemia. Etomidate and ketamine are frequently used to induce anesthesia in hemodynamically compromised patients, because these drugs are less likely to cause hypotension than thiopental or propofol. However, CNS excitation and increased ICP have been associated with these drugs, respectively, and they may not be appropriate for many neurosurgical patients. Ketamine should be avoided because of its known ability to increase cerebral metabolism, CBF, and ICP.
Airway Management
Developmental changes in airway anatomy have a significant impact on management of the pediatric airway. Nasotracheal tubes may be best suited for situations when the patient will be prone, because orotracheal tubes can kink at the base of the tongue when the head is a flexed and result in airway obstruction. The timing of tracheal extubation may be challenging after neurosurgical procedures. Infants, particularly those with Chiari malformation (Cochrane et al., 1990
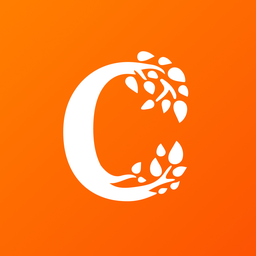
Full access? Get Clinical Tree
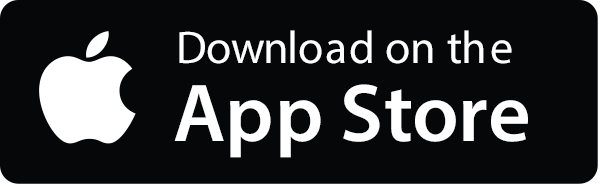
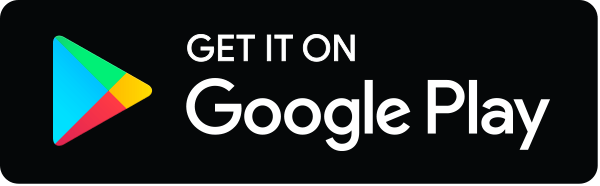