CHAPTER 18 Anesthesia for General Surgery in the Neonate
Surgical procedures during the neonatal period impose significant physiologic perturbations on the infant. With increasing regularity, diseases of adult life have been linked to events in early development (in utero, newborn, infancy, or childhood). For example, intrauterine growth retardation has been linked to an increased risk for a variety of adult disorders, including coronary heart disease, stroke, hypertension, type II diabetes, and even psychiatric illness (Barker and Osmond, 1986a; Barker, 1995, 2006; Ravelli et al., 1998; Singhal et al., 2003, 2004; Barker et al., 2005; Mittendorfer-Rutz et al., 2007). In addition, early postnatal and childhood nutrition and obesity have been associated with metabolic disorders such as dyslipidemia and glucose intolerance, growth abnormalities, and cardiovascular disease in adults (Huang et al., 2007; Mikkola et al., 2007; Rakow et al., 2008). These adult disorders with pediatric foundations were initially based on Barker and Osmond’s epidemiologic study (1986b). However, subsequent research has expanded his original “thrifty-phenotype” hypothesis (i.e., survival of the undernourished fetus demands that nutrition be directed to vital organs such as the brain, resulting in insulin resistance in other tissues such as muscle and the pancreas) to include a “developmental plasticity” theory (Hales and Barker, 2001; Gluckman et al., 2005; McMillen and Robinson, 2005). These initial reports have spawned an entire focal point for research and a model termed the developmental origins of health and disease (Gluckman et al., 2005; Gillman et al., 2007; Silveira et al., 2007). Recently, the increased risk for cardiovascular and metabolic disease has been extended to include preterm infants, both those who are appropriate for gestational age (AGA) and those who are small for gestational age (SGA) (Hofman et al., 2004a, 2004b; Mikkola et al., 2007).
Anesthetic pharmacology in the neonate
Increasing evidence indicates that the physiologic response of neonates to painful stimuli is similar to that of adults (see Chapter 15, Pain Management). The response of the sympathetic nervous system to noxious stimulation includes tachycardia and hypertension, which in the setting of abnormal cerebral autoregulation predisposes the infant with a low birth weight (LBW) to intraventricular hemorrhage and possibly pulmonary hypertension. The goal during anesthesia is to avoid pain and its cardiovascular and neurologic consequences. The response of newborns to opioids and potent inhalation agents is variable, and meticulous titration is critical for neonates undergoing surgery, both to avoid cardiovascular collapse and to maintain acid-base balance and also to eliminate awareness and pain.
Pharmacokinetics and Pharmacodynamics
Pharmacokinetics and pharmacodynamics of drugs are affected by anatomic factors relating to body composition and distribution of water, as well as physiologic factors such as metabolism (i.e., hepatic biotransformation); protein binding; and pathologic factors (e.g., disease, anesthesia, and surgery) (see Chapter 7, Pharmacology of Pediatric Anesthesia). Maturational changes in distribution of total body water, tissue composition, and organ function contribute to the unique response of the newborn and young infant to various drugs. In early fetal development, water constitutes approximately 94% of body weight. As gestation continues, the total body water decreases so that at 32 weeks, 80% to 90% of body weight is water, and at term, total body water is approximately 70% to 75% of body weight. Adult proportions of fluid to body weight (55%) are reached between the ages of 9 months and 2 years. The distribution of water between the extracellular and intracellular compartments also changes during fetal growth. Extracellular water (interstitial fluid plus plasma volume) decreases from 60% of body weight at the fifth month of fetal life to approximately 45% at term. Intracellular water increases from 25% in the fifth month of fetal life to 33% at birth; therefore, the extracellular fluid compartment of the newborn is equal to or greater than the intracellular fluid space. In adults, the intracellular and extracellular fluid compartments are approximately 40% and 20% of body weight, respectively. Because the plasma component of the extracellular fluid compartment remains at approximately 5% of body weight throughout life, it is the interstitial water that is greater in infancy (40%) and declines to 10% to 15% in the adult (Friis-Hansen, 1971).
Age-dependent changes in body composition also occur. At term, fat constitutes 11% of body weight. Fat content doubles by 6 months of age and is approximately 30% at 1 year. Teenage girls remain with approximately 20% to 30% fat, whereas fat in teenage boys decreases to 10% to 15% fat. Moreover, the composition of fat tissue changes with age. Fat of the newborn may contain as much as 57% water and 35% lipids; adults have 26% water and 71% lipids (Friis-Hansen, 1971). Skeletal muscle comprises 25% of total body mass in a full-term newborn compared with 43% in an adult.
The binding of drugs to serum proteins depends on several factors, including the concentration of protein, the number of binding sites on these proteins, and the affinity of the binding sites. The concentration of total serum protein, albumin, and α1-acid glycoprotein is lower in early infancy and reaches adult levels by approximately 1 year of age (Pacifica et al., 1986). Albumin primarily binds acidic drugs; α1-acid glycoprotein binds basic drugs. The concentration of these two proteins and their binding affinities are deficient in the newborn (Piafsky and Woolner, 1982).
The primary organ for drug biotransformation is the liver, but the kidneys, intestines, lungs, and skin also have minor roles. Hepatic oxidation, reduction, and hydrolysis (nonsynthetic, phase I reactions) mature rapidly, achieving adult rates by 6 months (Niems et al., 1976). Drugs metabolized via this cytochrome P450-dependent monooxygenase system include phenobarbital and phenytoin. Conjugation reactions (synthetic, phase II reactions) convert drugs into more polar compounds to facilitate renal excretion. These systems also mature postnatally.
The renal excretion of drugs is a function of glomerular filtration rate (GFR), active secretion, and passive reabsorption. GFR and secretion increase in an age-dependent manner. Renal blood flow and GFR increase dramatically during the first postnatal week and more gradually during the next several months, and adult performance is achieved at approximately 6 to 12 months of age (Arant, 1978; Hook and Bailie, 1979). Tubular secretory and reabsorptive capacity also mature postnatally (Fetterman et al., 1965).
Inhaled Anesthetic Agents
Infants have a higher incidence of cardiovascular instability and cardiac arrest during induction of inhalational anesthesia than do older persons (Rackow et al., 1961; Friesen and Lichtor, 1982, 1983; Morray, 2000, 2002; Murat et al., 2004). This untoward effect of potent inhalational agents can be attributed to several factors, including faster equilibration, rapid myocardial uptake in infants, the increased anesthetic requirement, and sensitivity of the neonatal myocardium. Infants attain a higher concentration of inhaled anesthetic agents in the heart and brain than do adults at the same inspired concentration. Moreover, the neonatal myocardium has a smaller fraction of contractile mass, and the magnitude and velocity of fiber shortening are less than in the adult myocardium. These factors and the increased anesthetic requirement, which is inversely related to age, all produce a higher incidence of adverse cardiovascular effects in infants.
The rate of rise of the alveolar concentration of an inhaled anesthetic depends on several factors: the inspired concentration, alveolar ventilation, and uptake. The greater the alveolar ventilation, the faster is the rate of rise of the alveolar concentration. This effect of alveolar ventilation is affected by the size of the functional residual capacity (FRC). Infants and children have an FRC similar to that of the adult, 30 mL/kg per minute. In contrast, alveolar ventilation is much higher in the infant (100 to 150 mL/kg per minute) compared with the adult (60 mL/kg per minute). This difference parallels the greater oxygen consumption of the infant. Thus, in the normal term newborn who weighs 3.0 kg, the ratio of alveolar ventilation to FRC is approximately 5:1, compared with the adult, in whom the same ratio is 1.5:1. As a result of this difference, the time constant of the inhaled anesthetic equilibrium for infants is much shorter than for the adult. Consequently, changes in concentrations of inspired gas are reflected rapidly in alveolar levels. In fact, it has been demonstrated that alveolar levels of inhalational anesthetic agents reach equilibrium faster in infants that in adults (see Chapter 7, Pharmacology of Pediatric Anesthesia).
Blood-gas partition coefficients of isoflurane, halothane, and sevoflurane did not differ in preterm infants compared with full-term infants but were lower than in adults. Only serum cholesterol correlated with the blood-gas partition coefficients (Malviya and Lerman, 1990). The blood-gas partition coefficient is an important determinant of solubility and therefore the rate of rise of the alveolar concentration of an inhaled agent.
The effect of age on the solubility of the inhaled agents in tissue is also important in determining the rate of rise of the alveolar concentration of the agent—the rate of anesthetic induction. Data by Lerman et al. (1986) are consistent with earlier work documenting that anesthetic solubility in brain, heart, liver, and muscle increases with age. An increase in solubility may prolong uptake, delay equilibration of the tissue partial pressure of anesthetic, and prolong the time of induction. Lerman and others found that the rate of increase in tissue anesthetic partial pressure, and therefore alveolar anesthetic partial pressure, is approximately 30% more rapid in newborns than in adults.
Minimal alveolar concentration (MAC) is an estimate of anesthetic requirement and changes with age (Fig. 18-1) (Katoh and Ikeda, 1987, 1992; LeDez and Lerman, 1987). In the original study, Gregory et al. (1969) reported that infants in the first 6 months of life had the highest MAC. In a later study, newborns were noted to require approximately 25% less halothane at MAC compared with infants who are between 1 and 6 months of age (Lerman et al., 1983) (see Chapter 7, Pharmacology of Pediatric Anesthesia).
Intravenous Anesthetics and Analgesics
Several studies have shown an increased sensitivity to and more prolonged effects of barbiturates and morphine in the neonate and young infant (Kupferberg and Way, 1963; Way et al., 1965). These features have been attributed in part to the immaturity of the blood-brain barrier, allowing faster and greater penetration and therefore higher concentration of these drugs in the brain.
In 1981, Robinson and Gregory reported that after a 10 mL/kg bolus of lactated Ringer’s solution, 30 to 50 mcg/kg of fentanyl was a safe anesthetic for premature infants undergoing ligation of a patent ductus arteriosus (PDA). Several years later, evidence was presented that infants who received fentanyl in combination with d-tubocurarine and nitrous oxide in oxygen had an improved perioperative course compared with those infants who did not receive analgesics (Anand et al., 1987).
Plasma levels of fentanyl are lower in infants vs. children vs. adults (newborns were not studied) after similar intravenous doses (Singleton et al., 1987). Gauntlett and others (1988) noted that clearance of fentanyl in newborns increased during the first few weeks of life. Elimination half-life and volume of distribution did not change. In a study of newborns who were administered continuous fentanyl infusions, Saarenmaa and others (2000) noted that plasma clearance correlated with maturity (gestational age) and weight, whereas Santeiro and others (1997) noted a correlation of clearance with postnatal age (Fig. 18-2). Koehntop et al. (1986) have shown a highly variable disposition and elimination of fentanyl in neonates. In addition, infants with increased intraabdominal pressure (e.g., omphalocele, gastroschisis, or septic ileus) appeared to have a further increase in the elimination half-life compared with infants undergoing repair of a PDA or myelomeningocele. Davis and others (1989) noted that the clearance of alfentanil in newborn premature infants was markedly reduced compared with older children (Fig. 18-3).
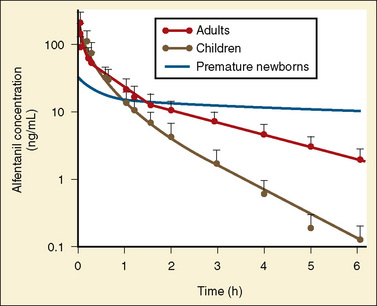
FIGURE 18-3 Age-related changes in alfentanil pharmacokinetics for premature infants, children, and adults.
(From Davis CM, Brando M: Pediatric pharmacology. In Greeley W, editor: Atlas of anesthesiology, vol 7, Philadelphia, 1999, Churchill Livingstone.)
Koren and others (1985) have shown that the elimination half-life for morphine (13.9 hours) in human neonates is markedly prolonged compared with that in older children and adults (2 hours). They also showed reduced clearance and higher serum concentration in neonates compared with those seen in older children after morphine infusion. Because of the large variability in clearance among neonates of different ages, the dose of opioids needs to be carefully titrated for each patient and each clinical setting.
Remifentanil is an ultrashort-acting opioid. Remifentanil is metabolized by plasma and tissue esterases, and its metabolism is independent of organ elimination. As a result, in neonates its pharmacokinetic profile is different from any other opioid. The remifentanil clearance rate in neonates is greater than in any other age group. Although its volume of distribution is large, its terminal elimination half-life does not change with age (Ross et al., 2001). Because remifentanil is metabolized in tissues and plasma, it does not accumulate, and consequently its context-sensitive half-time is flat (i.e., the time for 50% reduction at the effect site is independent of drug duration). Thus, remifentanil may be an ideal drug for anesthetic use in neonates.
Animal studies have shown a decreased median effective dose (ED50) for thiopental in the early weeks of life. Also, arousal in newborn rats occurred at lower brain levels of thiopental than in adult rats (Mirkin, 1975). Although this may suggest that lower doses of thiopental are needed in young neonates, studies by Jonmarker and others (1987) suggest the contrary. Similarly, ketamine requirements are greater (mg per kg of body weight) in infants than in older children (Lockhart and Nelson, 1974). Ketamine has been shown to produce apnea in infants with increased intracranial pressure (Lockhart and Jenkins, 1972). Ketamine produces hypertension and tachycardia, which some anesthesiologists have taken advantage of in caring for infants and children with congenital heart disease, cardiovascular instability, or both.
Propofol is commonly administered to infants. Propofol clearance is dependent on hepatic blood flow (high extraction coefficient) with subsequent metabolism and glucuronidation. In population pharmacokinetic analysis, Allegaert et al. (2007) noted that postmenstrual age and postnatal age were predictive covariants for clearance and that developmental maturation occurs within the first two weeks of life (Fig. 18-4).
Midazolam is an extensively used drug in both full-term and preterm infants and is metabolized by the P450 3A subfamily. Midazolam clearance in preterm infants is less than in children and older infants, and it may reflect the pattern of CYP3A4 ontogeny (Fig. 18-5). DeWildt et al. (2001), in a study of 24 preterm infants, found no relationship between age (postconceptual, gestational, or postnatal) and midazolam clearance. Of note was that in infants exposed postnatally to indomethacin, plasma clearance was higher and the volume of distribution was larger than in those infants not exposed to indomethacin. Because CYP3A4 expression is actuated during the first week after birth regardless of gestational age at birth, the decrease in midazolam clearance probably represents a decrease in CYP3A activity.
Muscle Relaxants
Developmental pharmacologic changes influence the requirements for muscle relaxants in infants and older children. Synaptic transmission is slow at birth, the rate at which acetylcholine is released during repetitive stimulation is limited, and neuromuscular reserve is reduced (Fig. 18-6). In addition, the reported sensitivity of infants to the effects of neuromuscular blocking agents has differed depending on whether drug administration was indexed to body weight or to body surface area. Because most neuromuscular blocking agents are distributed in the extracellular space and the extracellular space is related to the body surface area, dosage requirements for neuromuscular blocking agents often correlate with surface area rather than with body weight. Neonates and infants have a higher sensitivity (lower ED50 and ED95) for most nondepolarizing blocking agents.
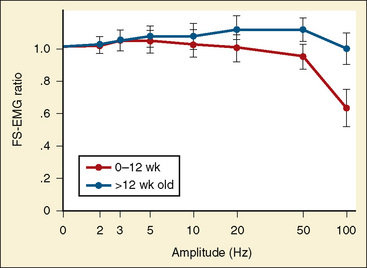
FIGURE 18-6 Tracings of the frequency sweep electromyogram (FS-EMG).
(From Crumrine RS, Yodlowski EH: Assessment of neuromuscular function in infants, Anesthesiology 54:29, 1981.)
Fisher et al. (1982) studied infants’ sensitivity to nondepolarizing muscle relaxants using the pharmacodynamic and pharmacokinetic properties of d-tubocurarine. These investigators determined the steady-state plasma concentration associated with 50% neuromuscular blockade (CPss50) and noted that infants had a lower CPss50 than older children. Because the volume of distribution of d-tubocurarine in infants is significantly larger than that in older children, the dose (mg per kg of body weight) required to achieve the same degree of neuromuscular blockade appeared the same for infants and older children. Although the pharmacokinetic data reveal similar clearance values for infants and older children, the infant’s larger volume of distribution and consequently longer elimination half-life suggest that infants need less frequent and smaller supplemental doses for continued neuromuscular relaxation. Although these data are specific for d-tubocurarine, the general principles can be extrapolated to other hydrophilic compounds that are primarily distributed to the “central compartment” (i.e., small volume of distribution).
Studies of rocuronium in infants and children have shown that onset time in small infants is faster, and the occurrence of 100% block at lower doses compared with older children suggests a greater potency in infants. In addition, rocuronium has an age-dependent difference in duration of action and in recovery following 0.45 mg/kg and 0.6 mg/kg doses (Fig. 18-7) (Driessen et al., 2000; Rapp et al., 2004).
General approach to intraoperative monitoring of the neonate
Thermal Protection
As already described, the newborn infant loses thermal protection after birth, so measures must be taken to protect against heat loss during surgery (Box 18-1):
Monitoring in the Operating Room
Circulatory Monitoring
A precordial stethoscope is a simple and effective means to assess the quality of heart sounds, rate, and rhythm, as well as breath sounds. A change in the intensity of heart sounds indicates a decrease in blood pressure and possibly cardiac output. Depending on the surgical procedure and the method of airway management (mask vs. laryngeal mask airway [LMA] vs. endotracheal tube), an esophageal stethoscope is an alternative monitor for noninvasive beat-to-beat cardiovascular monitoring. Although the sensitivity of the stethoscope has been discussed, the simplicity and accuracy of the precordial and esophageal devices to monitor heart tones and breath sounds during surgery involving pediatric patients cannot be denied (Hubmayr, 2004).
In most cases, the blood pressure can be accurately monitored with an automated device based on oscillometry (e.g., Dinamap) or ultrasonic flow (e.g., Arteriosonde) if the appropriate-sized cuff is available. Cuff inflation of the automatic devices should cycle no more frequently than every 3 to 4 minutes to avoid ischemia to the arm (Waugh and Johnson, 1984). Systolic blood pressure measurements correlate with the circulating blood volume and therefore are essential to monitor and guide fluid and blood replacement. Another alternative system is a Doppler ultrasonic transducer, which has a characteristic sound that decreases in intensity with a decrease in blood pressure.
An indwelling arterial cannula allows repeated blood sampling for cardiopulmonary and biochemical evaluation. A 22- or 24-gauge cannula can be inserted percutaneously or by cutdown into a variety of sites, including the radial, dorsalis pedis, or posterior tibial arteries. Before the insertion of the catheter, the adequacy of circulation to the hand should be assessed by applying a modified Allen’s test (Brodsky, 1975). The axillary artery is rarely cannulated. In general, the umbilical artery can be cannulated in the first 4 to 7 days of life, but this is usually avoided after the first 2 days of life because of the risks of infection and vascular emboli. The umbilical catheter tip can be placed high (T10 to T12) or low, above the bifurcation of the aorta and below the level of the renal arteries (L4, L5). The placement of the catheter must be confirmed by a radiograph (Fig. 18-8).
The risk for retinopathy of prematurity necessitates meticulous monitoring of oxygen saturation in the neonate, especially the very low–birth-weight (VLBW) infant. Most neonatologists recommend adjusting the inspired oxygen to maintain oxygen saturation between 90% and 95%, depending on the underlying medical status, gestational age, hemoglobin, and postnatal age (i.e., quantity of HgF). Of importance, if blood is shunting right-to-left through a PDA, the oxygen saturation measured in the lower extremities or umbilical artery (postductal site) does not reflect the oxygen saturation in the retinal vessels (preductal site). To enable simultaneous monitoring of both preductal and postductal oxygen saturation, two pulse oximeters are placed—one on the right hand (preductal) and one on a lower extremity (postductal). During right-to-left shunting through the PDA, the preductal oxygen saturation is higher than the postductal value, and the difference depends on the amount of shunting. If blood is shunting right-to-left only via the foramen ovale or other intracardiac sites (e.g., ventriculoseptal defect), the preductal and postductal oxygen saturation are equal (see Chapters 34, and 23, Respiratory Physiology in Infants and Children, Cardiovascular Physiology, and Anesthesia for General Abdominal, Thoracic, Urologic, and Bariatric Surgery).
A central venous catheter may be indicated to administer blood, fluid, total parenteral nutrition (TPN) and medications, and to monitor central venous pressure (CVP). Using the Seldinger technique, a catheter can be inserted percutaneously into the subclavian, internal jugular, external jugular, or femoral veins. In emergencies or situations of difficult venous access, the umbilical catheter can be inserted and passed into the right atrium (Fig. 18-8). Its location must be verified by x-ray or by a CVP tracing. Umbilical vein catheters have been associated with portal vein thrombosis. All central venous catheters are associated with significant morbidity, including thrombosis, emboli, and infection. Central venous catheters in the LBW infant have additional risks, from malpositioning and from the disruption of venous flow (ratio of the size of vessel to the size of the catheter is low).
Ventilatory Monitoring
Manual ventilation has been proposed as a technique to allow the anesthesiologist to continuously sense changes in compliance of the chest and airways. However, Spears and others (1991) noted that manual ventilation can be extremely unreliable for sensing changes in airway compliance. In addition to monitoring heart sounds, the precordial or esophageal stethoscope is a simple system to monitor ventilation and quality of breath sounds. Peak airway and end-expiratory pressures should also be measured. End-tidal carbon dioxide devices (mass spectrometers or infrared analyzers) are now the “standard of care” to continuously monitor the adequacy of respiratory exchange. These devices provide a breath-to-breath level of carbon dioxide tension, and the waveform of this measurement can provide information about rebreathing, ventilator disconnection, suspected air embolism, and hypermetabolic states (see Chapter 10, Equipment).
The pulse oximeter provides a precise, continuous readout of the hemoglobin oxygen saturation. During the first 1 to 2 weeks of life and without transfusion of autologous blood, the oxygen dissociation curve of HgF is shifted to the left of the adult curve so that hemoglobin saturation of 95% to 97% corresponds to an arterial oxygen tension (Pao2) of 52 to 77 mm Hg, assuming a Pao2 at 50% hemoglobin saturation (P50) of 19 mm Hg. The hemoglobin saturation should be correlated with an arterial partial pressure of oxygen (Po2) measurement to ensure valid interpretation of oxygen saturation data in the OR (see Chapter 3, Respiratory Physiology in Infants and Children).
Minimal-access surgery in the neonate
The development and refinement of endoscopic tools, optical technology, and surgical skills have allowed even the most complex neonatal procedures, such as congenital diaphragmatic hernia (CDH), tracheoesophageal fistula (TEF), congenital cystic adenomatoid malformation, and lobar emphysema, to be routinely accomplished with minimal-access surgery (Georgeson, 2003; Al-Qahtani and Almaramhi, 2006; Ponsky and Rothenberg, 2008). The field of minimal-access surgery continues to evolve and expand into other arenas such as robotic techniques, “stealth surgery” (or “scarless surgery”), and natural orifice transluminal endoscopic surgery (NOTES) (Dutta and Albanese 2008; Dutta et al., 2008; Isaza et al., 2008).
Endoscopic surgical techniques improve surgical conditions and produce better outcomes for patients. Improved technology has created endoscopic tools and video equipment that produce a magnified image and consequently better visualization of the surgical field. In patients with TEF the thoracoscopic approach improves the surgeon’s view, because the fistula is seen perpendicular to its connection to the membranous trachea; consequently the surgeon may more easily identify the exact site for ligation (Fig. 18-9) (Holcomb et al., 2005; Rothenberg, 2005b).
In addition to better visualization, minimally invasive surgery has also been associated with shorter postoperative recovery periods and decreased pain, inflammation, and scarring (Fujimoto et al., 1999). With less trauma to tissues, fluid therapy may be reduced as well. In some specific procedures, such as repair of a TEF, the avoidance of a posterior-lateral thoracotomy may prevent thoracic nerve damage, winged scapula, shoulder girdle weakness, fused ribs, chest wall asymmetry and muscular/soft tissue developmental abnormalities, and scoliosis (Jaureguizar et al., 1985; Rothenberg, 2005a;). In abdominal surgery, the use of minimally invasive techniques may decrease the incidence of adhesive injury after laparoscopy. This may be especially relevant in the newborn, in whom adhesive bowel obstruction after laparotomy ranges between 1% to 2% per year (Ponsky and Rothenberg, 2008).
Minimal-access surgery in neonates also presents challenges and limitations. The small working space and small size of the newborn, coupled with the unique aspects of neonatal physiology, make thoracoscopic surgery and one-lung ventilation difficult. There is a learning curve to minimally invasive surgery. Procedure times, operative morbidity, and conversion rates to open procedures vary among centers and surgeons (Ponsky and Rothenberg, 2008).
Efficient and safe endoscopic surgery requires both surgical and biotechnical expertise, as well as highly skilled OR and anesthesia teams (Kalfa et al., 2007). Because of these technical challenges, the OR times in the early learning phase may be markedly prolonged compared with operating times for the open procedures (Rothenberg, 2005a; Tsao and Lee, 2005; Nguyen et al., 2006; Kalfa et al., 2007).
Laparoscopy Background
These hemodynamic changes from insufflation, positioning, and hypercarbia are of minimal significance in a euvolemic, healthy adult, but the risks may become significant in patients with underlying cardiac disease (Grabowski and Talamini, 2009). Data documenting cardiorespiratory responses to laparoscopy in infants have been reported, but only about studies involving the use of noninvasive tools, and they differ from some of the results reported in healthy adults.
Using a transesophageal ultrasonic probe inserted after induction of general anesthesia in 12 healthy boys (23 ± 5 months of age), Gueugniaud et al. (1998) noted that pneumoperitoneum (10 mm Hg) decreased aortic blood flow and stroke volume and increased systemic vascular resistance. No effects on mean arterial pressure or end-tidal CO2 were noted. Of importance, these patients remained supine (no reverse Trendelenberg positioning).
Manner et al. (1998) reported the pulmonary effects of pneumoperitoneum in 10 patients between the ages of 1 and 15 years who were undergoing a variety of laparoscopic procedures (intraperitoneal pressure, 12 mm Hg). In this study, the patients’ pulmonary compliance decreased (17% with the reverse Trendelenberg position, 28% with the reverse Trendelenberg position plus insufflation); peak inspiratory pressure increased (25% with the reverse Trendelenberg position, 30% with the reverse Trendelenberg position plus insufflation) and end-tidal CO2 tension (Petco2) concentration increased from 33 to 42 mm Hg at maximum insufflation pressure. In 17 infants (younger than 10 months) who were undergoing a variety of procedures that required at least 30 minutes of laparoscopy but experienced no change in position, Bannister et al. (2003) reported similar findings. Patients were divided into two groups: those weighing less than 5 kg (intraabdominal pressure less than 12 mm Hg), and those weighing more than 5 kg (intraabdominal pressure, less than 15 mm Hg). Again, peak inspiratory pressure increased (18%) and correlated with insufflation pressure, and dynamic compliance decreased (48%). Tidal volume decreased 33% and oxygen saturation fell 2% to 11% in 41% of the patients. Ventilation was adjusted to maintain Petco2 within 10% of baseline, and 20 adjustments were reported. Neither heart rate nor blood pressure changed with insufflation or ventilatory adjustments, and significant changes from baseline status were noted at insufflation pressures of 10 mm Hg and greater but not at pressures of 5 mm Hg. Similar minimal hemodynamic effects of low-pressure pneumoperitoneum have been documented in young children elsewhere (DeWaal and Kalkman, 2003).
Of note, inducing a pneumoperitoneum in the Tredelenburg position during gynecologic surgery in adults decreases the distance between the endotracheal tip and the carina as well as the tracheal length (Kim et al., 2007). Because the distance between the larynx and the carina is markedly less in the infant, this phenomenon may be of critical importance in the newborn undergoing laparoscopy.
Renal function is affected by pneumoperitoneum. However, data have primarily been acquired in the adult population. In adults, decreased urine production has been attributed to the decreased glomerular filtration rate and renal perfusion from the direct effects of pneumoperitoneum (e.g., compression of the renal artery, the inferior vena cava, or the parenchyma) or from secondary effects (e.g., decreased cardiac output and release of mediators) (Rosin et al., 2002). Renal blood flow and renal function decrease during pneumoperitoneum; the effects on blood flow are pressure dependent and exacerbated in the head-up position, but they return to normal after deflation (Demyttenaere et al., 2007).
The effect of pneumoperitoneum on intracranial pressure has been studied in adults. Although the newborn’s cerebrovascular physiology and autoregulatory responses differ from those of the adult, newborns with limited autoregulatory responses may have more profound cerebral vascular responses from increased intraabdominal pressure. In adult animals, intraabdominal pressures of 15 and 25 mm Hg—but not 5 mm Hg—affected intracranial pressure, similar to findings by Rosenthal et al. (1998) (Rosin et al., 2002). Bloomfield et al. (1997) created models that linked intraabdominal, intrathoracic, and intracranial pressures. As with other studies, pleural, peak inspiratory, right atrial, and pulmonary arterial pressures increased as intraabdominal pressure increased above 10 mm Hg. The increases became especially significant at intraabdominal pressures of 25 mm Hg. At the same time cardiac index decreased, but systemic vascular resistance increased and blood pressure remained stable. Increased intracranial pressure accompanied the rise in intraabdominal pressure; however, these changes reversed when the abdomen was decompressed. Of significance, the increase in pleural and intracranial pressures with increased intraabdominal pressure was prevented in a group of swine with the chest opened (e.g., median sternotomy and pleuropericardotomy). However, in spite of the open chest, cardiac index decreased and systemic vascular resistance increased. The investigators suggested that the acuity of the increase in intrathoracic pressure secondary to an abrupt rise in intraabdominal pressure acutely increased central venous pressure, preventing cerebral venous outflow. When they prevented the effect of intraabdominal pressure on the pleural pressure (open chest), venous and intracranial pressures did not change.
The absence of predictable autoregulation of cerebral perfusion in the newborn combined with the significant risk of injury secondary to oxygen and positive pressure ventilation may have unique implications for the anesthetic management of the newborn during either laparoscopy or thoracoscopy. Although indirect monitoring of cerebral blood flow is available (e.g., near-infrared spectroscopy, or NIRS), ideally a reliable, continuous method to accurately monitor cerebral perfusion pressure would be of critical benefit in this setting (Brady et al., 2007).
Laparoscopy and Thoracoscopy in the Newborn
The effects of insufflation during both laparoscopy and thoracoscopy are dramatic in the newborn. The impact of increased intrathoracic pressure and hypercarbia can profoundly influence the transitional circulation. Changes in patient oxygen saturation, hypotension, interruption of the procedure, and hypercarbia are common in the newborn (Kalfa et al., 2005, 2007; Krosnar and Baxter, 2005).
Insufflation in the newborn also decreases core body temperature. In 50% of newborns, the postoperative core temperature was less than 36° C. A long operative time (insufflation for more than 100 minutes) was associated with lower postoperative core body temperature (Kalfa et al., 2005).
Inaccurate end-tidal CO2 values are common during laparoscopy, especially in the setting of cyanotic heart disease (Laffon et al., 1998; Wulkan and Vasudevan, 2001). At the same time, absorption of CO2 from the peritoneum seems to be age-dependent (McHoney et al., 2003). The total amount of insufflated CO2 and elimination of CO2 correlated with age. Younger patients also demonstrated a short period of increased CO2 elimination after desufflation, which may be related to increased venous return after the decrease in intraabdominal pressure.
Because one-lung ventilation is challenging to achieve and at times is not tolerated by a newborn, many pediatric surgeons suggest that insufflation of the chest with low flow of CO2 (1 to 2 L/min, peak pressure of 4 to 6 mm Hg) in the 30- to 45-degree prone position induces adequate lung collapse (Rothenberg, 2002; 2005b; Holcomb et al., 2005).
In spite of the myriad of critical physiologic challenges, endoscopic procedures have been conducted successfully in high-risk infants with conditions such as hypoplastic left heart syndrome, complex cyanotic heart disease, and patients with single ventricle physiology (Mariano et al., 2005; Rice-Townsend et al., 2007; Slater et al., 2007).
Specific neonatal surgical lesions
Abdominal Wall Defects: Gastroschisis and Omphalocele
Gastroschisis and omphaloceles are the most common abdominal wall defects (ectopia cordis and cloacal and bladder extrophy are less common), but they are still rare, with an incidence of approximately 1:10,000 and 1:4000 to 7000 births (Paidas et al., 1994). Some have suggested that both ethnic and geographic origins affect the incidence, but overall the lesions seem sporadic (Mann et al., 2008).
Currently, fetal ultrasounds are routinely conducted to screen for high-risk conditions. For example, nuchal translucency and biochemical markers (free β-subunit of human chorionic gonadotropin and pregnancy-associated plasma protein A) are now measured to screen for aneuploidy or cardiac and other anomalies (Souter and Nyberg, 2001; Weiner et al., 2007). An abnormal value on screening prompts an evaluation that includes a detailed fetal ultrasound; in the case of abdominal wall defects, ultrasound confirms the presence of a lesion in approximately 95% of cases after as few as 10 to 14 weeks’ gestation (Mann et al., 2008). The in utero diagnosis allows planned delivery at a medical center with resources for high-risk obstetric, surgical and anesthetic, and neonatal care.
Although omphalocele and gastroschisis appear to be similar in gross physical appearance, these lesions are distinct from each other. An omphalocele is a central defect of the umbilical ring; the abdominal contents herniate into the intact umbilical sac unless the sac ruptures in utero (Fig. 18-10). The umbilical cord is also inserted into the sac, which contains an internal peritoneal membrane and external amniotic membrane. The lesion has a fascial defect of more than 4 cm (fewer than 4 cm is often considered an umbilical hernia) and often as large as 10 to 12 cm. The sac often contains the stomach, loops of the small and large intestines, and in about 30% to 50% of cases, the liver. A gastroschisis, on the other hand, is an abdominal wall defect that usually occurs to the right of the umbilical cord, with evisceration of bowel through the defect (Fig. 18-11). This lesion is usually 2 to 5 cm in diameter, and in most cases there are only small and large bowel present. In rare cases the liver may exit through the abdominal wall defect as well. The bowel is exposed to the intrauterine environment with no sac, causing its loops to become matted, thickened, and often covered with an inflammatory coating or peel. Whether this exudative peel is secondary to a specific inflammatory pathway or just an effect of amniotic fluid is unclear. The umbilical cord is normal and separate from the defect. When the testes exit along with the bowel, cryptorchidism occurs with gastroschisis (Lawson and de La Hunt, 2001; Weber et al., 2002).
Embryology
Although experimental models have been described, the embryologic etiology of these lesions is not completely understood (Correia-Pinto et al., 2001). Omphalocele has been described as a failure of the cephalic, lateral, and caudal folds to fuse (closure of the exocelomic space) and as abnormal fusion and differentiation of myotomes to form abdominal wall musculature (7 to 12 weeks of gestation). That is, the abdominal cavity is primarily underdeveloped. During weeks 7 to 12 of development, the midgut elongates and herniates into the umbilical cord. By week 12, the abdominal cavity is large enough for the developing gut to exit the cord and reenter the abdomen. Some believe that the simple failure of this return to the abdomen is a developmental arrest that results in an omphalocele and a small abdominal cavity. The abdominal cavity is small only because the gut remains in the umbilical sac.
Gastroschisis is considered to be an earlier embryologic event, resulting from an abnormality of the development of the right omphalomesenteric artery or right umbilical vein, which in turn results in ischemia to the right paraumbilical area. More recently, abdominal wall defects have been ascribed to an abnormal relationship between cell proliferation and planned cell death (apoptosis) at the critical embryonic folding period (Robinson and Abuhamad, 2000). Inadequate mesoderm development may contribute to dysplastic abdominal wall growth. This underdeveloped site is commonly found just to the right of the umbilicus and ruptures with increased pressure of the growing intraabdominal organs. Similar defects in other areas may produce other lesions, such as extrophy of the bladder.
Gastroschisis is usually an isolated lesion, but recent reports raise the possibility of familial occurrence (Yang et al., 1992; Torfs et al., 1994). In utero exposure to acetaminophen, aspirin, and pseudoephedrine has been associated with an increased incidence of gastroschisis (Werler et al., 2002; Baerg et al., 2003). In the case of either an omphalocele or gastroschisis, rotation of the gut is incomplete in utero, resulting in various “malrotation” phenotypes. Intestinal atresias are common, especially in patients with gastroschisis.
Although neither gastroschisis nor omphalocele is considered to be familial, 50% to 75% of infants with an omphalocele have other anomalies, and 20% to 30% have chromosomal abnormalities (Robinson and Abuhamad, 2000; Weber et al., 2002; Mann et al., 2008). Only 15% to 20% of infants with gastroschisis have associated malformations, and only rarely is there a complex pattern of malformation (Stoll et al., 2008). For example, Beckwith-Wiedemann, Rieger’s, and prune belly (Eagle-Barrett) syndromes are associated with omphaloceles, as are trisomies 13, 15, 18, and 21. If the omphalocele contains only bowel and if oligo- or polyhydramnios are present, the likelihood of an associated chromosomal abnormality increases. Others have noted that omphaloceles that contain liver and other viscera are more likely to have cardiac, renal, or limb anomalies, whereas the smaller omphaloceles are more likely to have gastrointestinal or central nervous system malformations (Mann et al., 2008). Syndromes of midline defects often include an omphalocele, and the lesion has been noted in the nonsyndromic multiple congenital anomalies (MCA) complex.
Single-gene mutations have been identified in omphaloceles with multiple anomalies, such as the filamin A, alpha (FLNA) gene in the otopalatodigital syndrome, which is characterized by abnormalities in the axial and appendicular skeleton plus extraskeletal anomalies such as omphalocele (Robertson, 2007). One syndrome, Pentalogy of Cantrell, includes an omphalocele, diaphragmatic hernia, sternal abnormalities, an ectopic and anomalous heart, and gene abnormalities at Xq25 to Xq26.1. Another syndrome (OEIS Complex) involving the lower abdomen includes omphalocele, bladder or cloacal extrophy, imperforate anus, colonic atresia, sacrovertebral anomalies, and meningomyelocele. No environmental or teratogenic associations have been proposed as etiologic causes. Allen et al. (2006) reported an association with assisted reproductive technology. Thus, identifying an abdominal wall defect in utero demands an intense evaluation for associated anomalies, which may be isolated, part of a recognizable malformation syndrome, or chromosomal. In addition, serial ultrasounds should be conducted to evaluate fetal growth and well being, as well as amniotic fluid volume. Of note, after 20 to 32 weeks’ gestation, a higher incidence of fetal demise has been reported. The mode of delivery for infants with abdominal wall defects remains controversial, but recent studies have shown that in gastroschisis and nongiant omphaloceles (i.e., liver extracorporeal), outcome after vaginal delivery is no different than with caesarean section (Henrich et al., 2008). The controversy centers on delivery with gastroschisis because of the unprotected viscera, but no advantages of cesarean section are consistently apparent when survival and incidence of complications are evaluated (Weber et al., 2002).
Preoperative Management
Normothermia should be maintained or achieved by preventing heat loss from the exposed viscera. A bowel bag may be used for this purpose (Towne et al., 1980). Emphasis should be placed on covering the lesion with gauze, nonadherent dressing, and a plastic cover rather than moist (saline-soaked) material, as moisture tends to exacerbate heat loss (Mann et al., 2008
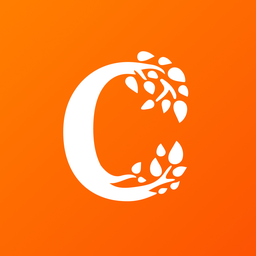
Full access? Get Clinical Tree
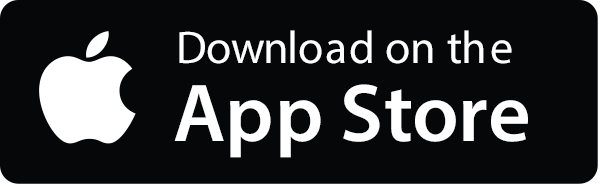
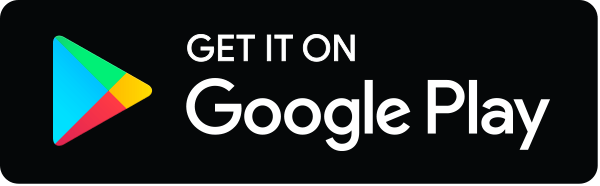