CHAPTER 19 Anesthesia for Fetal Surgery
Fetal interventions present a unique therapeutic opportunity. With advances in medical imaging technology, fetuses can be screened for anatomic congenital defects early in the course of a pregnancy. Although postnatal therapy is adequate for many fetuses, some congenital defects will not allow a successful transition to extrauterine life. Other birth defects may result in death in utero or serious disability after birth (Harrison, 1996). The logistics of fetal interventions can be quite challenging, but the ideas behind many of the treatments are conceptually quite simple. Some examples of interventions include bypass of fetal airway obstruction that would be fatal at birth, resection of a lung lesion causing hydrops fetalis, and repair of a myelomeningocele to minimize long-term disability (Table 19-1). Prenatal therapy opens new territory by making the fetus an active recipient of a given intervention. While the fetus is actively treated, the mother, by necessity, also receives medical care. Because of the high risk of these procedures, patient selection is an important consideration. The mothers must be at a sufficiently low anesthesia risk, and they must be highly motivated to comply with frequent follow-up and activity restrictions. Providing anesthesia for these patients requires a thorough understanding of maternal, fetal, and placental physiology. Familiarity with minimally invasive and open procedures and an understanding of the diseases amenable to fetal therapy are important. A list of key terms and acronyms is given in Box 19-1.
Box 19-1 Acronyms and Glossary
Amnioreduction: Percutaneous needle drainage of excess amniotic fluid
CDH: Congenital diaphragmatic hernia
CHAOS: Congenital high airway obstruction syndrome
EXIT: Ex utero intrapartum therapy
HLHS: Hypoplastic left heart syndrome
Polyhydramnios: Excess amniotic fluid, which may be a sign of fetal pathology
TRAPS: Twin reversed arterial perfusion sequence
Diseases
Nonimmune hydrops threatens the lives of both the fetus and mother (van Selm et al., 1991). The aptly named maternal mirror syndrome is a state of maternal edema that mirrors that of the fetus. The pathophysiology of this process is unclear, but it may involve a maternal inflammatory response to shedding of debris from a hydropic placenta (Redman and Sargent, 2000). In contrast to preeclampsia, the mother is hemodiluted rather than hemoconcentrated, and the fetus, by definition, must show signs of hydrops (Vidaeff et al., 2002). Pulmonary edema secondary to the mirror syndrome may be life threatening, and treatment of maternal mirror syndrome involves either delivery of the fetus or treatment of the cause of the hydrops (Heyborne and Chism, 2000; Pirhonen and Hartgill, 2004; Livingston et al., 2007).
Complicated Multiple Gestations
Monozygotic twin gestations are at increased risk for complications. If a monozygotic cell mass splits within 3 days of fertilization, a dichorionic diamniotic twin gestation should result. If the mass splits later than 13 days after fertilization, conjoined twins will result. If the cell mass splits between days 3 and 13, the twins will be “conjoined” to varying degrees at the level of the placenta and placental vessels (Lewi et al., 2003). These conjoined placental vessels may result in a net flow of blood from one twin (donor) to the other twin (recipient). The donor will suffer from hypovolemia, oligohydramnios, and growth restriction, whereas the recipient will suffer from hypervolemia, polyhydramnios, hydrops, and heart failure (Luks et al., 2005). Ultrasound and fetal echocardiographic changes may be used to describe the severity of disease (Quintero et al., 1999; Rychik et al., 2007). This process, twin-twin transfusion syndrome, places both twins at risk for preterm delivery, death, and neurologic disability.
Traditional treatment of twin-twin transfusion syndrome involves serial amnioreduction to relieve the polyhydramnios. This may decrease the risk of preterm labor and may allow better perfusion of the donor twin; however, this therapy does not target the physiologic problem, which arises from the connected placental vessels (Lewi et al., 2005). Newer therapies target the placental vessels, employing percutaneous placement of endoscopes into the amniotic fluid to allow visualization and ablation of the connected placental vessels (Fig. 19-1). Minimally invasive fetoscopic laser ablation of the vessels has been shown to be superior to amnioreduction in a randomized clinical trial (Senat et al., 2004). Another trial showed no difference between amnioreduction and laser ablation, but there were differences in the studies that do not allow direct comparison (Crombleholme et al., 2007). More research is needed to fully define the role of minimally invasive laser therapy and amnioreduction in the treatment of twin-twin transfusion syndrome.
Twin reversed arterial perfusion sequence (TRAPS) is a similar clinical entity in which one of the twin cell masses may be acardiac, acephalic, or both. The normal or “pump” twin is at risk for death from high-output heart failure because it must supply cardiac output for itself and the acardiac cell mass (Tan and Sepulveda, 2003). In these cases, selective feticide of the acardiac cell mass will allow the normal pump twin to survive. This is accomplished by occlusion of blood flow to the umbilical cord of the acardiac mass either by bipolar cautery or radiofrequency ablation (Tsao et al., 2002). If the twins are monochorionic and monoamniotic, the umbilical cord of the acardiac cell mass must also be divided to prevent cord entanglement and death of the normal twin. These umbilical cord occlusions are accomplished with minimally invasive techniques, using ultrasound or fetoscopic guidance.
Neurologic
Fetal therapies for prenatally diagnosed hydrocephalus and myelomeningocele (MMC) have been described. Results for in utero treatment of hydrocephalus with ventriculoamniotic shunts have not been encouraging, and this therapy is no longer actively studied or offered (Manning et al., 1986; Bruner et al., 2006). However, in utero closure of MMC defects is more promising. This closure involves accessing the midgestation fetus via maternal laparotomy and hysterotomy (Fig. 19-2). The MMC is repaired, and uterine and abdominal incisions are closed. Pregnancy is continued with the goal of delivering as close to term as possible.
The rationale for in utero closure of MMC is based on animal models of fetuses with these lesions, where it appears that prolonged bathing of neurologic elements in the amniotic fluid worsens the neurologic outcome (Meuli et al., 1995; Meuli et al., 1996). In human studies, closure of fetal MMC decreases the need for postnatal ventriculoperitoneal shunting and the incidence of hindbrain herniation and Chiari malformation. Motor function may be somewhat improved, and cognitive behavioral testing does not appear to be adversely affected (Bruner et al., 1999; Tulipan et al., 1999; Johnson et al., 2003; Johnson et al., 2006). A randomized trial sponsored by the National Institutes of Health (NIH) is currently enrolling patients who are randomized either to standard postnatal closure of MMC or to in utero closure of MMC. Enrollment in the trial is, at this time, the only avenue for this surgery.
Airway
Airway obstruction is life threatening in the postnatal period, especially when airway patency cannot be established immediately on delivery by intubation. Extrinsic causes of airway obstruction include cystic hygroma and oral teratoma. Cystic hygroma is a type of lymphatic malformation that may develop in the neck, resulting in large, fluid-filled cystic masses (Fig. 19-3). Teratomas are germ cell tumors composed of tissues foreign to their normal location (Fig. 19-4). Polyhydramnios may result from an inability of the fetus to swallow amniotic fluid. Intrinsic causes of airway obstruction include laryngeal cysts, webs, and atresia. The spectrum of disease due to intrinsic compression has been called congenital high airway obstruction syndrome (CHAOS) (Hedrick et al., 1994; Lim et al., 2003).

FIGURE 19-3 A newborn who underwent aspiration of a cystic hygroma and subsequent intubation during an EXIT procedure.
(Photo courtesy of Alan W. Flake, MD, Children’s Hospital of Philadelphia.)
Airway obstruction may be fatal in utero. This is not immediately intuitive, because the placenta serves as the organ of respiration, but complete airway obstruction will not allow egress of lung fluid into the amniotic space. This fluid will collect, and massive pulmonary hyperplasia may result. The fetal diaphragm may be flattened or even project into the abdominal space (Fig. 19-5). The intrathoracic pressure is presumably increased and will impede cardiovascular filling and function, resulting in nonimmune hydrops and fetal demise (Mong et al., 2008). If hydrops is not present, fetal airway obstruction is well served by the ex utero intrapartum therapy (EXIT) procedure. The EXIT procedure for a near-term fetus involves a maternal laparotomy and hysterotomy, exteriorization of the fetus, and securing of the fetal airway before clamping and division of the umbilical cord. After the cord is divided, the fetus is delivered and given to a neonatology team for further stabilization. If hydrops is present and the lungs are immature, midgestation tracheal decompression may be a treatment option (Kohl et al., 2006; Kohl et al., 2009).
Lung Lesions
Lung lesions that may be diagnosed in utero include congenital diaphragmatic hernia (CDH), congenital cystic adenomatoid malformation (CCAM), and bronchopulmonary sequestration (BPS). CDH has been a target for in utero therapy for decades. Results with both open and minimally invasive techniques have thus far been equivalent to those with optimal postnatal therapy. Thus, the risk-benefit analysis would support postnatal therapy. However, efforts at refining minimally invasive therapy continue and may prove to be of benefit in the future (Harrison et al., 1997; Harrison et al., 2003; Deprest et al., 2005; Doné et al., 2008; Peralta et al., 2008).
The management of prenatally diagnosed CCAM is instructive because its natural history is variable (Fig. 19-6). CCAM results from an overgrowth of terminal bronchioles. These masses do not participate in gas exchange, may predispose to infection and malignancy, may cause mediastinal shift and cardiac compression, and may result in hypoplasia of the remaining lung tissue. Some CCAMs will grow and some will shrink—each at unpredictable, variable rates. Regular prenatal assessment is required. Lesion composition (microcystic or macrocystic) and size have varying physiologic effects. Taking pulmonary maturity into consideration, the treatment of CCAM may occur in early, mid, or late gestation. Therapies may be minimally invasive or require open fetal surgery. Compromise results mostly from mass effect or fluid collection, resulting in a tamponade physiology. Solitary lesions with a large single cyst may be treated minimally invasively with one-time or serial needle aspiration. Ultrasound-guided minimally invasive placement of a thoracoamniotic shunt may negate the need for serial procedures in large, cystic lesions.
In contrast to large lesions with a single cyst, microcystic lesions are not amenable to aspiration or shunting. If a midgestation fetus with immature lungs has significant physiologic compromise, an in utero fetal pulmonary lobectomy may be pursued. After the lobectomy is completed, the fetus is returned to the uterus for further growth and development (Adzick et al., 2003). If the fetus is late in gestation and the lesion is causing distress or may interfere with neonatal resuscitation, a delivery via the EXIT procedure can be undertaken, with fetal thoracotomy and pulmonary lobectomy before umbilical cord clamp (Hedrick et al., 2005). If the mass is asymptomatic, the child can be delivered normally, and a postnatal pulmonary lobectomy can be electively scheduled.
Cardiac Disease
Most of the therapies for congenital cardiac malformations are minimally invasive and catheter based, with most of the reported literature describing fetal aortic valvuloplasty for treatment of severe aortic stenosis—a possible precursor to hypoplastic left heart syndrome (Tworetzky et al., 2004; Marshall et al., 2005; Wilkins-Haug et al., 2006). In utero atrial septoplasty (Fig. 19-7) has also been described for patients with hypoplastic left heart with an intact or highly restrictive atrial septum (Marshall et al., 2008). Pulmonary atresia with an intact ventricular septum may also be amenable to fetal cardiac intervention, as this is the “right-sided” equivalent of hypoplastic left heart syndrome (Tworetzky and Marshall, 2004). Earlier surgical techniques involved a maternal laparotomy and exteriorization of the uterus. The necessary needles and catheters were then inserted through an otherwise intact uterus, and subsequently guided through the fetal chest wall and myocardium to the chambers or vessels of interest. Currently, an even less invasive approach to the fetal heart is performed. The approach is entirely percutaneous: through the maternal abdomen, uterus, and fetal chest wall. Great care and skill come into play as the trajectory of the needles, catheters, and wires is planned. Outcomes from fetal cardiac interventions have been encouraging but are not definitive yet.
Bladder Outlet Obstruction
Fetal urinary tract obstruction (Fig. 19-8) may result in a host of complications, such as pulmonary hypoplasia, renal dysplasia, bladder dysfunction, renal failure, skeletal abnormalities, and abdominal wall muscular abnormalities (Harrison et al., 1981; Harrison et al., 1982). Patient selection for therapy is challenging, because the disease process must be severe enough to warrant a prenatal surgical intervention, but not so far advanced that the renal damage is irreversible (Cendron et al., 1994). Fetal evaluation is quite involved and includes ultrasound examination of the urinary tract, measurement of amniotic fluid volumes, and analysis of fetal urine electrolytes and proteins (Crombleholme et al., 1990). Minimally invasive therapy may involve needle decompression of the bladder, vesicoamniotic shunting, or ablation of posterior urethral valves. Open surgery for bladder marsupialization or ureterostomies has also been reported (Crombleholme et al., 1988). Decompression may reduce the severity of oligohydramnios and the resulting pulmonary hypoplasia, but it may not allow recovery of renal function. Placement of a vesicoamniotic shunt may be technically successful, but the shunt may become dislodged. Bladder function may still not be optimal, as the fetal bladder is not exposed to cyclic filling and emptying. Open fetal surgery has been performed, but the risks and benefits must be carefully considered (Crombleholme et al., 1988).
Sacrococcygeal Teratoma
Sacrococcygeal teratoma is a germ cell tumor arising from the coccyx (Fig. 19-9). Prenatal diagnosis is associated with much higher mortality than postnatal diagnosis (Flake, 1993). In utero, the teratoma places the fetus at risk of hydrops and death from high-output heart failure (Bond et al., 1990). Serial echocardiography is used to monitor the cardiac status of the fetus, and ultrasound is used to monitor tumor growth (Tran et al., 2008). The tumor may cause a vascular steal phenomenon, where much of the fetal cardiac output goes to supplying the tumor instead of the fetus. This is evidenced by increased flow in the descending aorta and increased diameter of the fetal inferior vena cava (Hedrick et al., 2004). The tumor may also rupture in utero, resulting in fetal anemia. Treatment may be minimally invasive in select cases of cystic tumors and involves aspiration of the cystic components. Therapy with radiofrequency ablation has not yielded consistent results (Paek et al., 2001; Ibrahim et al., 2003). In certain cases, midgestation debulking of the tumor may be attempted. An EXIT procedure for resection of a massive sacrococcygeal teratoma has also been performed. For large tumors, at the very least, a planned cesarean delivery is necessary, with provisions for immediate resection if necessary.
Physiology
Maternal
Sensitivity to anesthetic agents increases during pregnancy. Early in gestation, the minimum alveolar concentrations (MACs) for isoflurane and for halothane are approximately 30% lower in pregnant women than in nonpregnant women (Gin and Chan, 1994; Chan et al., 1996). CSF volume decreases, and the epidural venous plexus becomes distended (Kerr et al., 1964). Pregnancy enhances the spread of local anesthetics in the spinal fluid (Hirabayashi et al., 1995b); in the epidural space, the spread of small dosages of local anesthesia is increased, but the spread of large dosages is about the same in pregnant and nonpregnant women (Kalas et al., 1966; Grundy et al., 1978). Increased dermatomal spread of epidural anesthetics is likely to result from increased nerve sensitivity, hormonal changes, reduced protein levels, and pH changes in the CSF (Flanagan et al., 1987; Hirabayashi et al., 1995a; Hirabayashi et al., 1996; Popitz-Bergez et al., 1997).
Plasma cholinesterase activity decreases during pregnancy, but this decrease does not clinically affect the dosing of succinylcholine. Pregnancy also increases sensitivity to nondepolarizing muscle relaxants. Onset time is faster for vecuronium, and recovery time is longer for rocuronium (Baraka et al., 1992; Puhringer et al., 1997).
Airway management in a pregnant woman is potentially more difficult than in a nonpregnant woman. Capillary engorgement of the airway mucosal tissues has multiple implications. Resultant airway narrowing forces downsizing of endotracheal tubes, and nasal intubation is likely to cause epistaxis. The potential for difficult intubation is increased and airway complications are a significant factor in anesthesia-related morbidity and mortality (Cormack and Lehane, 1984; Samsoon and Young, 1987; Pilkington et al., 1995; Ezri et al., 2001; Ross, 2003; Munnur et al., 2005; Rudra et al., 2006; Goldszmidt, 2008). Respiratory and metabolic changes place the mother at increased risk of hypoxia. Oxygen consumption increases by as much as 60% (Spatling et al., 1992) and functional residual capacity (FRC) begins to decrease in the second trimester, reaching 80% of the nonpregnant volume at term (Alaily and Carrol, 1978). FRC decreases further in the supine position. Tidal volume and minute ventilation increase by 45% at term, and Paco2 decreases to 30 mm Hg by the second trimester (Kelman and Templeton, 1975; Templeton and Kelman, 1976; Alaily and Carrol, 1978). The risk of pulmonary edema increases after open fetal surgery (DiFederico et al., 1998). This increased risk may be secondary to decreased plasma protein concentration, decreased colloid oncotic pressure, or medications used to terminate premature labor, or a combination of these (Mendenhall, 1970; Wu et al., 1983). In addition, surgical manipulation of the uterus and hysterotomy may release factors that increase pulmonary vascular permeability (DiFederico et al., 1998).
Pregnancy is a high cardiac output state. Heart rate and stroke volume increase as early as the first trimester, and at term cardiac output is increased approximately 50% from nonpregnant values (Laird-Meeter et al., 1979; Hunter and Robson, 1992). Cardiac output increases even more during labor and immediately postpartum (Robson et al., 1987). Systemic vascular resistance decreases by about 20% secondary to vasodilation and the addition of the placenta, which is a low-resistance vascular circuit (Clark et al., 1989). Supine hypotension may occur because of compression of the inferior vena cava by the gravid uterus. This compression results in a decrease of right atrial pressure in the supine position (Kerr, 1965). Compression of the aorta is most pronounced in the supine position and becomes negligible in the full lateral position (Eckstein and Marx, 1974). Human studies suggest a reduced response to arterial vasoconstriction by alpha agonists but an increased sensitivity to venous constriction (Nisell et al., 1985). Thus, any pressor response may be more likely to result from increased venous return and increased stroke volume. Plasma volume increases relatively more than red blood cell volume increases, and hemoglobin concentrations decrease in pregnancy.
The pregnant patient is at increased risk for pulmonary aspiration of gastric contents. Hormonal changes and displacement of the stomach with resultant reduction of tone in the lower esophageal high-pressure zone may allow reflux of gastric contents (Ulmsten and Sundstrom, 1978). Intragastric pressure is highest in the third trimester. Gastric emptying of solids and liquids is not slowed during pregnancy, but gastric emptying does slow down during labor (Davison et al., 1970; O’Sullivan et al., 1987; Macfie et al., 1991; Sandhar et al., 1992; Whitehead et al., 1993; Chiloiro et al., 2001; Wong et al., 2002). Although gastric acid production has not definitively been shown to be greater in pregnancy, acid aspiration is still a major concern.
Fetal
Fetal physiology is as complex as maternal physiology. Logistical considerations necessitate some extrapolation from animal studies. Neurologic pathways for cortical transmission of noxious stimuli in humans are still developing into the third trimester (Lee et al., 2005). Fetal sensitivity to volatile anesthetic agents is increased. With both isoflurane and halothane, the anesthesia requirement of fetal lambs is lower than that of a pregnant ewe (Gregory et al., 1983; Bachman et al., 1986). Perception and processing of pain are controversial, but noxious stimuli will elicit a physiologic response in the human fetus, as evidenced by increases in cortisol and β-endorphin, and decreases in the pulsatility index of the fetal middle cerebral artery (Fisk et al., 2001). These physiologic responses are attenuated by administration of fentanyl directly to the fetus. The pharmacokinetics and pharmacodynamics of fentanyl in the fetus remain to be studied.
The cardiopulmonary physiology of the fetus is not dependent on the lungs, because the placenta acts as the organ of respiration. A major role of the lungs in utero is the production of amniotic fluid. The lung epithelium actively secretes fluid, which fills the lungs. Excess fluid passes out the trachea and into the oropharynx. This fluid is then either swallowed or expelled into the amniotic space and becomes a component of amniotic fluid. Fluid in the lungs causes distention and allows lung development (Olver and Strang, 1974; Perks and Cassin, 1985; Harding and Hooper, 1996). Restriction of egress of this fluid from the lungs results in pulmonary hyperplasia, whereas continuous drainage results in pulmonary hypoplasia (Alcorn et al., 1977).
When the pulmonary and systemic circulations are in a series, as in a normal adult heart, cardiac output can be quantitatively described by either the left or the right ventricular output. Because the fetus has a parallel circulatory system, fetal cardiac output is measured and reported as the sum of both the left and right ventricular output, or combined cardiac output (CCO). Echocardiographic studies have found that a normal human fetus has a CCO of 425 to 550 mL/kg per minute, with the right ventricle contributing 60% to 70% of the total output (De Smedt et al., 1987; Mielke and Benda, 2001; Rychik, 2004). The fetal myocardium has a greater proportion of noncontractile elements and is also stiffer with impaired relaxation compared with adult myocardium (Friedman, 1972; Rychik, 2004). Functioning close to the upper limit of the Starling curve, increases in preload provide only minimal, if any, incremental increases of stroke volume and cardiac output (Gilbert, 1980). Variation in heart rate provides a relatively greater contribution to variation in cardiac output. This lack of response to preload has been attributed to poor compliance of the myocardium, but it may also result from extrinsic compression of the fetal heart that is relieved with aeration of the lungs and clearance of lung fluid (Grant, 1999; Grant et al., 2001).
The blood volume of a fetus varies over gestation. At 16 to 22 weeks, blood volume of the fetoplacental unit has been estimated to be 120 to 162 mL/kg of fetal weight (Morris et al., 1974; Nicolaides et al., 1987). At 31 weeks, blood volume has been reported as 93 mL/kg of fetal weight (Nicolaides et al., 1987). It is important to note that about two thirds of the blood volume is contained on the placental side of the fetoplacental unit (Barcroft and Kennedy, 1939; Yao et al., 1969). A 16-week fetus weighs approximately 100 g, at 22 weeks 430 g, and at 31 weeks 1500 g.
Studying hemostasis is challenging because of the rapid evolution of the coagulation system in the fetal and neonatal period. The fetus produces coagulation factors independently of the mother, and these factors do not cross the placenta (Cade et al., 1969). The plasma concentrations of these proteins increase with increasing gestational age (Reverdiau-Moalic et al., 1996). A fetus at 19 to 23 weeks of gestation has a mean prothrombin time (PT) of 32.5 seconds with an International Normalized Ratio (INR) of 6.4 and a mean activated partial thromboplastin time (aPTT) of 168.8 seconds. At 30 to 38 weeks of gestation, mean PT is 22.6 seconds with an INR of 3.0 and an aPTT of 104.8 seconds (Reverdiau-Moalic et al., 1996).
While in utero, fetal temperature is closely linked to maternal variations in temperature. Fetal tissue is more metabolically active than adult tissue, and fetal heat production results in a fetal-to-maternal gradient of 0.5 °C. Excess heat flows from the fetus and is dissipated by the mother. After birth, an increase in heat production is required for homeostasis with the loss of the maternal “heat clamp,” and with new evaporative and convective heat losses. The sympathetic nervous and endocrine systems generally activate and regulate this heat production. Shivering and nonshivering thermogenesis take a primary role in the generation of heat for the newborn (Power et al., 2004). A fetus removed from the uterus during open surgery has a similar need for increased heat production as a newborn, but it cannot increase its production adequately. Both fetal shivering and nonshivering thermogenesis responses are largely absent in utero if the umbilical cord is patent (Gunn et al., 1986). Maintenance of normothermia in a fetus exposed during open fetal surgery can be challenging, with a lack of shivering and nonshivering thermogenesis, immature skin barriers, and increased evaporative losses.
Uteroplacental Blood Flow
The fetus depends on intact uteroplacental blood flow and patent umbilical vessels for respiration and nutrition. Uterine blood flow, a surrogate for fetal oxygen delivery, correlates with fetal umbilical venous Po2 (Skillman et al., 1985; Bilardo et al., 1990). Human uterine blood flow at term is extrapolated to be 700 mL/min (12% of cardiac output), compared with 200 mL/min in the nonpregnant uterus (Thaler et al., 1990).
Uterine blood flow is directly related to uterine perfusion pressure (the difference between uterine arterial pressure and uterine venous pressure) and inversely related to uterine vascular resistance (Box 19-2). Of note for fetal surgical procedures, maternal hypotension, aortocaval compression, and uterine contractions will decrease uterine blood flow. The effect of vasopressors, vasodilators, and anesthetic agents on uterine blood flow is variable because these agents affect uterine arterial pressure and uterine vascular resistance at the same time. Ephedrine has been suggested as the vasopressor of choice in obstetrics, as it may not cause as much uterine arterial vasoconstriction as phenylephrine. Several studies have shown no dramatic clinical differences in neonatal outcome and lend slightly more support to phenylephrine to support maternal blood pressure. When phenylephrine is compared with ephedrine to support blood pressure during cesarean section, newborn pH measurements are statistically but not clinically different (Thomas et al., 1996; Lee et al., 2002
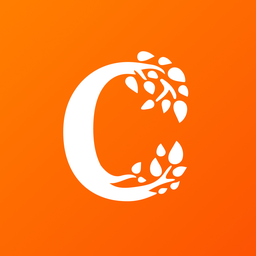
Full access? Get Clinical Tree
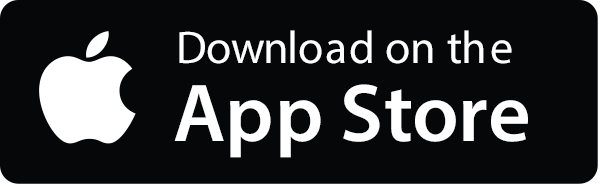
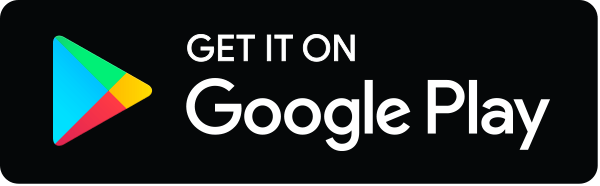