31 Advanced Bedside Neuromonitoring
Monitoring Neurologic Status
The analytical approach to a patient with a neurologic problem is a process that requires the physician to have a specialized anatomic and physiologic knowledge of the nervous system. Daily evaluation of neurologic and mental status should be included in the neuromonitoring protocol. Function of pyramidal and extrapyramidal systems, status of cranial nerves, function of cerebellum and spinal cord whenever possible, and any trend in change of neurologic status should be recorded for every patient as part of neuromonitoring. In critically ill patients, however, such a complete neurologic evaluation can sometimes be unreliable or impossible owing to the use of sedatives and the need for intubation and ventilatory support as part of the medical treatment of the neurologic problem. Along with the neurologic examination, information about vital signs and key laboratory values should be available at the bedside in a 24-hour record sheet. A handheld pupillometer (ForSite NeurOptics Automated Infrared Pupillometer, NeurOptics Inc., Irvine, California) is a new technology that may reduce observer variability in the neurologic examination. Infrared quantitative pupillometry can produce accurate, reproducible pupillary measurements which are clearly superior to those obtained manually at the patient’s bedside by even an experienced nurse or physician.1 An important limitation of this device is that assessment is quite challenging in patients with altered mental status, in patients with periorbital or scleral edema, and in uncooperative patients. Ambient light and physiologic factors may also affect the measured pupillary characteristics.2 A recent study using this device reported good reliability when correlating the pupillary constriction velocity as a predictor of intracranial pressure (ICP) elevation in neurosurgical patients. More clinical experience is needed before including the pupillometer as a standard of care.3
The Glasgow Coma Scale (GCS) is used as a standardized scale for recording neurologic status in the ICU. The Glasgow outcome scale has been the standard outcome tool for neurocritical care. New tools such as the Neurological Outcome Scale for TBI (NOS-TBI) have been adapted for traumatic brain injury (TBI) patients from the National Institutes of Health Stroke Scale (NIHSS) and potentially could serve as a tool for initial stratification of injury severity and prediction of long-term outcome.4
Intracranial Pressure and Cerebral Perfusion Pressure
Normal resting ICP in an adult is less than 10 mm Hg. A sustained ICP value greater than 20 mm Hg is considered clearly abnormal. Mild to moderate intracranial hypertension is considered to be present when ICP is between 20 and 40 mm Hg, and values greater than 40 mm Hg are considered severe, life-threatening intracranial hypertension. In the 2007 Guidelines for the Management of Severe TBI, an ICP threshold above 20 to 25 mm Hg was adopted as a level III recommendation to initiate treatment to reduce ICP in patients with severe life-threatening head injury.5
Cerebral perfusion pressure (CPP) is the difference between the mean arterial blood pressure (MAP) and ICP. Under normal physiologic conditions, a MAP of 80 to 100 mm Hg and an ICP of 5 to 10 mm Hg generate a CPP of 70 to 85 mm Hg.6
Under normal circumstances, the brain is able to maintain a relatively constant CBF of approximately 50 mL per 100 g/min over a wide range of CPP (60 to 150 mm Hg).7 This process called pressure autoregulation is a complex regulatory mechanism involving both myogenic and metabolic components. Following injury, the ability of the brain to pressure autoregulate can be impaired, and CBF is often dependent on CPP.
The indications and thresholds for monitoring of CPP remain controversial. The current recommendation in TBI is to target CPP values within the range of 50 to 70 mm Hg.8 CPP values less than 50 mm Hg increase the risk of cerebral ischemia and hypoperfusion, while therapies required to maintain CPP values greater than 70 mm Hg have been associated with an increased risk of acute respiratory distress syndrome (ARDS).9 Some recent evidence suggests that the status of cerebral autoregulation should play a role in therapeutic decisions. If pressure autoregulation is intact, a CPP-directed therapy may be used with a greater chance for a favorable outcome. However, if pressure autoregulation is impaired, ICP-guided therapy may be of more benefit.10 The on-line correlation between ICP and MAP (pressure reactivity index [PRx]) or between middle cerebral artery blood flow velocity and MAP (Mx) is used to assess the status of pressure autoregulation and direct management in some critical care units.11,12
Intracranial Pressure Monitoring Devices
The current gold standard for ICP monitoring is the ventriculostomy catheter or external ventricular drain (EVD), which is a catheter inserted in the lateral ventricle, usually via a small right frontal burr hole. This ventricular catheter is connected to a standard pressure transducer via fluid-filled tubing. The external transducer must be maintained at a specific level. The reference point for ICP is the foramen of Monro, although in practical terms, the external auditory meatus is often used as a landmark. EVDs measure global ICP and have some useful advantages over other ICP monitors, including the ability to perform periodic in vivo external calibration and therapeutic CSF drainage and CSF sampling. When intracranial mass lesions or ventricular effacement due to swelling are present, EVD placement may be difficult even for the most experienced neurosurgeon. Intraventricular catheters are also associated with the highest rate of infection among the ICP monitors. Several microtransducer-tipped ICP monitors are now available on the market for clinical use (e.g., Camino ICP monitor, Codman microsensor, and Neurovent-P ICP monitor). These microtransducers can be inserted in the subdural space or directly into the brain parenchyma. Neurovent microsensors incorporating three monitoring variables (ICP, brain tissue oxygen partial pressure, and temperature) are now available; however, current clinical data with this device are limited.13 Although there are fewer risks of infection and intracranial hemorrhage with these catheters, the main disadvantage is that none can be calibrated in vivo; after preinsertion calibration, they may exhibit zero drift (degree of difference relative to zero atmospheres) over time.14
The Spiegelberg ICP monitor, which incorporates pneumatic technology, has been recently introduced. This device uses a small air pouch balloon at the end of a catheter to sense changes in pressure and automatically does in vivo calibration. A novel “lab-on-a-tube” intraventricular catheter was recently developed for multimodal neuromonitoring in patients with TBI; it provides real-time ICP, glucose, oxygen, and temperature monitoring and in situ therapeutic CSF drainage.15
Noninvasive ICP monitoring methods have been developed with the aim to reduce the risks associated with invasive monitors. Displacement of the tympanic membrane has been used to determine temporal changes in ICP.16 Recent data suggest that optical detection of cerebral edema using either broadband halogen illumination or a single-wavelength near-infrared (NIR) laser diode may allow earlier detection of cerebral edema compared with the traditional ICP monitors.17 Transcranial Doppler (TCD) pulsatility index and magnetic resonance imaging (MRI) of the optic nerve sheath have been used to provide a noninvasive estimate of ICP.18–21 Measurement of peripapillary retinal nerve fiber layer thickness with optical coherence tomography is a noninvasive quantitative technique to monitor evolution of papilledema as a predictor of intracranial hypertension.22 So far, none of these methods have provided accuracy sufficient to replace invasive ICP monitors.
ICP Waveforms
Typically, the normal ICP waveform consists of three arterial components superimposed on the respiratory rhythm. The first arterial wave is the percussion wave, which reflects the ejection of blood from the heart transmitted through the choroid plexus in the ventricles. The second wave is the tidal wave, which reflects brain compliance; and finally, the third wave is the dicrotic wave that reflects aortic valve closure. Under physiologic conditions, the percussion wave is the tallest, with the tidal and dicrotic waves having progressively smaller amplitudes. When intracranial hypertension is present, cerebral compliance is diminished. This is reflected by an increase in the peak of the tidal and dicrotic waves exceeding that of the percussion wave (Figure 31-1).
Complications
The incidence of infection for ICP devices is reported to be 1% to 27%,23 depending on the type of ICP monitoring device. A recent study investigated the complications with use of an ICP fiberoptic device (Camino) alone and in combination with an external ventricular drain (EVD) catheter; the infection rate was 1.8% and 7.9%, respectively.24 Several other factors have been identified that may affect the risk of EVD infection: use of prophylactic parenteral antibiotics; presence of other concurrent systemic infections; presence of intraventricular or subarachnoid hemorrhage; duration of monitoring; open skull fracture, including basilar skull fractures with CSF leak; leakage around the ventriculostomy catheter; and repeated flushing of the EVD. Routine exchange of ventricular catheters and prophylactic antibiotic use for EVD placement is not recommended to reduce infection rate.25 However, placement of ICP monitors should be done under the most sterile possible conditions, minimizing excessive manipulation and flushing. Although there is evidence that antibiotic-impregnated catheters may decrease rates of infection, more trials should be conducted to evaluate the beneficial effect on clinical outcome.26
Jugular Venous Oxygen Saturation
Placement of a jugular venous oxygen saturation (SjvO2) catheter involves retrograde insertion into the internal jugular vein of a catheter equipped with an oxygen sensor at the tip. The internal jugular vein catheter is similar to the type used for CVP monitoring but is directed cephalad into the jugular bulb.27 The tip of the catheter must be placed above the C1-C2 vertebral bodies to avoid contamination with blood coming from the facial vein. Correct positioning of the catheter can be confirmed with a lateral skull x-ray (Figure 31-2). The incidence of complications related to the SjvO2 catheter is low but includes carotid artery puncture, hematoma formation, infection, thrombosis, and increase in ICP that may arise during catheter insertion or due to prolonged monitoring.
The development of in vivo reflectance oximetry using fiberoptic catheters has allowed continuous monitoring of SjvO2 without the need of continuous blood sampling, except for calibration purposes.28 Changes in SjvO2 should be confirmed by measuring the oxygen saturation in a blood sample withdrawn from the jugular venous catheter, and the catheter should be recalibrated if the difference is more than 4% to increase the duration of good-quality records.27,29 Risks of intravascular catheters and possible contamination with noncerebral blood being monitored might be eliminated in the future with a promising noninvasive technique of monitoring the superior sagittal sinus. The technique detects ultrasound waves generated in tissue as pulsed NIR radiation is absorbed, resulting in thermoelastic expansion of the irradiated volume.30
Side of Jugular Catheterization
The choice of side for jugular bulb monitoring remains a debate.31,32 The jugular venous catheter can be placed on the side with the worst pathology or the side where the internal jugular vein circulation is dominant. The dominant internal jugular vein is the side with the largest vein by ultrasound imaging or by ICP response to venous compression. If the strategy is to use SjvO2 as a monitor of global oxygenation, then cannulating the dominant jugular vein is logical because it is most representative of the whole brain. However, if the strategy is to identify the most abnormal oxygen saturation, then the side with the most severe injury should be cannulated.33
Normal Jugular Venous Oxygen Saturation
SjvO2 reflects the global balance between cerebral oxygen delivery (supply) and the cerebral metabolic rate of oxygen (demand). When arterial oxygen saturation, hemoglobin concentration, and the hemoglobin dissociation curve remain stable, SjvO2 generally parallels changes in CBF. Values defining the normal range of SjvO2 are still debated but are usually considered to be 50% to 54% for the lower range and 75% for the upper range.28,34,35 Multiple pathologic clinical scenarios may cause an increase or decrease in SjvO2 values (Table 31-1). A large number of studies have assessed the role of jugular venous saturation monitoring in patients with severe TBI. In 1992, Sheinberg et al. demonstrated that single or multiple episodes of jugular venous desaturation were associated with a higher mortality rate.36 However, high SjvO2 values indicating low cerebral oxygen extraction have also been associated with poor outcome,35 and an elevated mean arteriojugular oxygen content difference has been associated with a better outcome.37 This apparent discrepancy where increased cerebral oxygen extraction is both associated with a higher mortality rate and a better neurologic outcome can probably be explained by specific circumstances in individual patients. Greater cerebral oxygen extraction means a higher cerebral metabolic rate (and better prognosis) so long as cerebral metabolic requirements are met.38 According to the most recent consensus for brain oxygen monitoring and thresholds, evidence supports a level III (SjvO2 <50%) recommendation for use of jugular venous oxygen saturation, in addition to the standard ICP monitors in the management of patients with TBI.39 Because SjvO2 provides only information of a global state of cerebral oxygenation, focal ischemic areas are not evaluated with this technique.
TABLE 31-1 Clinical Conditions Associated with Alterations in SjvO2 Values
Increased SjvO2 | Restricted oxygen diffusion or extraction due to neuronal infarction or inflammation Decreased cerebral metabolism Increased systemic oxygen supply due to hyperoxia Hyperemia |
Decreased SjvO2 | Local or systemic hypoperfusion (e.g., intracranial hypertension, shock or prolonged hypotension, vasospasm) Decreased systemic oxygen supply (e.g., low PaO2) Increased cerebral metabolism or oxygen extraction (e.g., seizures, fever) |
Local or Regional Monitoring
Transcranial Doppler Flow Velocity and Flow Volume
Cerebral vasospasm is a major cause of disability after subarachnoid hemorrhage (SAH) and TBI, with similar incidence in both groups.40 The incidence of critical regional CBF reductions due to vasospasm are seen progressively when flow velocities above 120 cm/sec are present by TCD examination.41 Angiography remains the gold standard for diagnosing cerebral vasospasm, but TCD ultrasonography gives a noninvasive alternative for daily bedside monitoring of the CBF dynamics. The Lindegaard ratio (middle cerebral artery-to-extracranial internal carotid artery flow velocity ratio) helps in differentiating vasospasm from hyperemia; vasospasm is considered to be present if the Lindegaard index is greater than 3:1.42 In hyperemia, flow velocity for both intracranial and extracranial vessels increases, whereas in vasospasm, high flow velocity is seen only in intracranial vessels, resulting in a high ratio.
TCD studies have high specificity for the confirmation of brain death. Brief systolic forward flow spikes with reversed or absent diastolic flow found bilaterally or in three different arteries are accepted TCD criteria for supporting the diagnosis of brain death.43
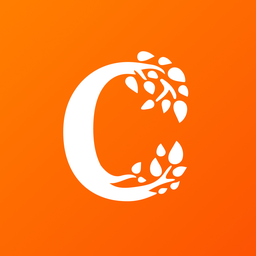
Full access? Get Clinical Tree
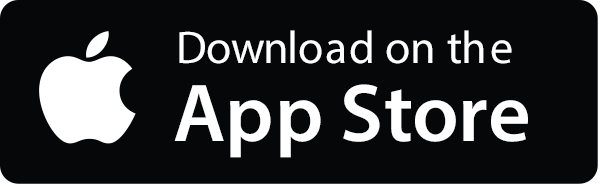
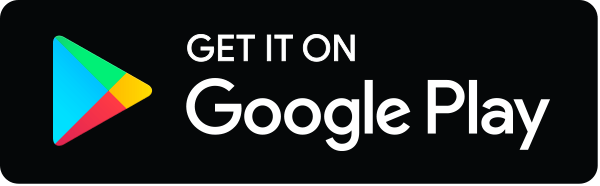