Addressing Respiratory Discomfort in the Ventilated Patient: Introduction
Although respiratory discomfort (dyspnea) is as common as pain in seriously ill patients,1 there is no formal requirement to assess and manage it, such as the “fifth vital sign” requirement for pain assessment and management.
Mechanical ventilation is the most frequently used life-support measure in the intensive care unit (ICU). It is also used during anesthesia and the initial phase of postanesthesia awakening, and at home in patients with chronic respiratory failure. Many patients who are mechanically ventilated are thus conscious (or even fully awake), yet they can experience rapid and important changes in their respiratory status and metabolic needs. It is thus not surprising that ventilated patients do experience respiratory discomfort. They can report and quantify it, and there are strategies to address it. In this chapter we (a) propose that respiratory discomfort be routinely assessed in ventilated patients, and (b) suggest some approaches to managing this discomfort with minimal sedation. Together, these could be termed patient-centered ventilation.2 There is, however, a paucity of studies directly addressing prevalence, outcomes, and mechanisms of dyspnea and its relief in acutely ventilated patients.
Occurrence and Sequelae of Respiratory Discomfort in Ventilated Patients
Although we lack large-scale studies on the prevalence of dyspnea in mechanical ventilation, there have been a few studies in which a small sample of acutely ventilated patients have been asked to rate dyspnea.3–8 In three studies the severity of dyspnea was stratified: In a combined total of 204 patients, 19% of the respondents reported moderate to severe discomfort.3,6,7 In one study, patients were asked to characterize the quality of their discomfort: 69% reported experiencing air hunger; 51% reported excessive work/effort. About a third of the foregoing patients experienced both air hunger and work/effort.7 In another study, 29% of ventilated patients recalled after their stay in the ICU that they had been moderately to extremely bothered by not getting enough air from the endotracheal tube, which we equate to air hunger.9 Many patients, however, were too heavily sedated to respond; many of these latter patients had probably been heavily sedated in an attempt to alleviate respiratory discomfort. This clearly indicates that there is a problem to address. As discussed below, heavy sedation is not necessarily an adequate solution and may be harmful.
There is a clear association between anxiety and dyspnea in patients.10–12 In laboratory subjects, experimentally induced air hunger produces more discomfort and more anxiety than tasks that require a great deal of respiratory work and effort.13 In critically ill patients, emotional reactions such as panic, anxiety, and fear are significantly correlated with patient–ventilator dyssynchrony.14 Dyspnea can cause anxiety, even in healthy subjects who experience dyspnea in a safe laboratory situation.13,15,16 Conversely, anxiety can cause dyspnea. This is true even in the absence of cardiopulmonary pathology; for example, dyspnea is the most common symptom in anxiety and panic disorders.17 Because of this reciprocal causation, the possibility of positive feedback exists in ventilated patients, with dyspnea causing anxiety, which then exacerbates the dyspnea. In a series of mechanically ventilated patients reporting dyspnea, multivariate analysis showed that the strongest statistical association with respiratory discomfort was with anxiety.7 In the subset of these patients whose dyspnea was lessened by changes in ventilator settings, anxiety was also reduced, suggesting that the dyspnea caused the anxiety.
Survivors of mechanical ventilation in the ICU often carry surprisingly dark recollections of the experience. A recent study reported that 15% of patients recalled feeling at risk of being murdered, 17% felt betrayed, 39% felt at risk of imminent death, and 55% felt they were being suffocated.18 Thus, it should not be surprising that posttraumatic stress disorder (PTSD) is now recognized as a common sequela of ICU experience.18–21 PTSD symptom scores in the post-ICU population are significantly correlated with duration of mechanical ventilation,19 and with recalled memories of respiratory distress.20 A number of reasons for these connections can be postulated, but the possibility cannot be ignored that inescapable dyspnea is one of the traumatizing events leading to PTSD in this population.
Assessment of Discomfort in the Ventilated Patient
The first step in addressing dyspnea is to determine how much and what kind of discomfort the patient is experiencing. A full assessment requires that the patient be alert enough to respond to simple direct questions regarding the intensity and quality of discomfort. In heavily sedated patients, the daily wake-up practiced in many ICUs22 provides an opportunity to assess the intensity of dyspnea and attempt to reduce it with nonpharmacologic approaches before evaluating the need for further sedation.
Several rating methods have been shown to be feasible in intubated and ventilated patients. Patients who are alert enough to respond but who cannot speak can provide information through simple number, word, or visual analog scales, similar to those routinely used in clinical pain assessment.3,5,23 Patients can point to the appropriate responses but in some cases the interviewer will need to say or point to responses in succession and stop when the patient indicates with a blink or other sign. The intensity scale may be combined ad hoc with a list of descriptors from which the subject can choose; descriptor lists often make the process easier for patients who find it difficult to describe unfamiliar internal sensations.24Table 55-1 shows a brief sample assessment procedure. More extensive and sophisticated assessment may be required for research purposes.25
Please indicate on this 10-point scale how your breathing feels:
|
Many caregivers rely on physical signs such as respiratory rate, heart rate, use of accessory muscles, synchrony with mechanical ventilation, diaphoresis, and facial expression. Recently, a systematized Respiratory Distress Observation Scale (RDOS) was developed and validated in spontaneously breathing subjects.26 This scale comprises measures of heart rate, respiratory rate, accessory muscle use, paradoxical breathing pattern, restlessness, grunting at end-expiration, nasal flaring, and fearful facial display. Some of these signs are likely to be altered by mechanical ventilation, so adaptation and validation of the scale for ventilated patients is needed. Signs can be very useful when patients are unable to communicate, but they can be misleading.5 Patients vary widely in their behavioral responses to discomfort; thus, as with pain, physical signs may overestimate or underestimate the degree of discomfort, and they give little information regarding its cause. Reliance on inconsistent physical signs was also a problem in pain assessment until accrediting organizations, such as Joint Commission on Accreditation of Healthcare Organizations (JCAHO), began to require more direct assessment and management of pain.27 Caregivers should ask the patient whenever feasible.
Dyssynchrony of patient and ventilator is associated with discomfort. It has been suggested that it is a cause of discomfort and conversely that it is a result of discomfort (both may be true). The interaction between modern ventilators and patients is a result of the interface between two complex control systems, and the resulting pressure and flow waveforms can be complex. Dyssynchrony is usually assessed qualitatively by visual inspection of waveforms, but some investigators are attempting to formally quantify dyssynchrony.28 When such methods have matured, they must be validated against subjective reports of patients. Again, we emphasize that when patients can report their discomfort, their reports should be weighed more heavily than physical signs. To date, the available studies of patient–ventilator synchrony have not measured its relationship to respiratory discomfort.
There are a number of circumstances under which a ventilated patient will be unable to communicate verbally with caregivers and where physical signs of respiratory distress will be difficult to interpret. A particularly difficult instance is the patient paralyzed with neuromuscular blockers. Neurophysiologic surrogates of dyspnea would be particularly useful in this setting. Although in theory one might be able to assess respiratory discomfort using functional magnetic resonance imaging of the brain,29 this is unlikely to become practical for clinical use in the foreseeable future.
Electroencephalography, however, may provide a more practical method. In normal volunteers under experimental conditions, preinspiratory potentials become apparent during noninvasive mechanical ventilation when respiratory discomfort is induced through the use of inappropriate ventilator settings.30 Preliminary observations in mechanically ventilated patients suggest that similar monitoring of respiratory-related electroencephalography activity could help detect respiratory discomfort in noncommunicant patients. This approach would allow a continuous surveillance that would be particularly useful in patients liable to experience frequent changes in respiratory status. The clinical utility of these techniques and the spectrum of their applicability are important research targets.
In addition, assessing the electromyographic activity of inspiratory muscles can be useful as a measure of respiratory drive, one of the determinants of respiratory sensation discussed in the air hunger section. There is experimental and clinical evidence that “ventilator fighting” or patient–ventilator dyssynchrony can be associated with visibly increased activity of inspiratory neck muscles, parasternal intercostals, and upper airway dilators including the tongue.31–33 Correlations between respiratory muscle activity and dyspnea have been observed.31,33 The clinical usefulness of respiratory muscle electromyography to assess and monitor respiratory discomfort is another likely research topic.
A particular patient’s discomfort may be caused by a mix of respiratory discomfort and nonrespiratory sensations, such as pain and nausea. Little is known about how these sum into total discomfort; in some cases, one noxious stimulus can occlude perception of another. The limited data available suggest that simultaneous dyspnea and pain can be separately scaled, and that the presence of one does not strongly affect the other, at least under controlled circumstances.34 Sensations of respiratory discomfort other than dyspnea (e.g., secondary to cough or irritation from the endotracheal tube) may also be present. Different forms of dyspnea such as air hunger and excessive work of breathing may be simultaneously present, and are thought to be additive.35 The problems of ventilated patients are therefore varied and complex; there is no single approach that will work in all patients.
Primer on Dyspnea
Several different mechanisms give rise to respiratory discomfort; the mechanism(s) operating in an individual patient will guide the treatment approach selected. The quality of discomfort is one guide to understanding which mechanisms are in play; other clues come from physical examination and measurement of ventilatory variables (see “Approach to the Ventilated Patient with Dyspnea”). There are at least three distinct qualities of uncomfortable breathing sensations: air hunger, effort or work, and tightness. These different forms of dyspnea are caused by different afferent mechanisms and are evoked by different physiologic stimuli.
Theories developed in the late 1960s and early 1970s attributed all dyspnea to excessive work or effort of breathing.36 Although work of breathing has since been disproved as the sole origin of dyspnea,37–39 this model still dominates thinking in the realm of clinical mechanical ventilation.40,41 Attempts to understand a patient’s discomfort based only on work of breathing will not be fully effective; in fact, extremely unpleasant dyspnea can be evoked in the absence of any respiratory work.
Air hunger is the conscious perception of the need for more air that is typically described by subjects as “not getting enough air,” “uncomfortable urge to breathe.” This is the sensation felt by normal subjects at the end of a long breathhold.15,38 Subjects comment that air hunger is a threatening or frightening sensation. Healthy subjects exposed briefly to air hunger in our laboratory have reported that “it’s a feeling…you’re going to die because you’re not getting enough air.” Even moderate air hunger is highly aversive if prolonged, with one subject commenting that “if I felt I had to live my life feeling like that I would jump out the window [commit suicide].” Quotes from patients with dyspnea can be remarkably similar: “When the shortness of breath was at its extreme I thought I was going to die,” and “…wouldn’t want to live if it continued.”42
Air hunger arises from stimulation of arterial chemoreceptors and from other drives to breathe; a rise in partial pressure of arterial carbon dioxide (PaCO2) or a fall in partial pressure of arterial oxygen (PaO2) will evoke air hunger.37,43–46 Air hunger is not caused by contraction of respiratory muscles; complete respiratory paralysis does not abolish or even diminish the air hunger response to CO2.37–39 It is hypothesized that air hunger is transmitted to the cerebral cortex by a corollary discharge of medullary respiratory center activity (also known as ventilatory drive). Such a corollary discharge has been described in the midbrain and thalamus of decorticate cats, presumably en route to the cortex.47–49 Although air hunger is stimulated by medullary drive, the two are not equivalent; other processes modify the translation of drive into the perception of air hunger in the cerebral cortex. Air hunger is associated with activation of the paralimbic cortex in humans, a region of the brain long associated with emotional responses and learning. The principal activation reported in studies of experimental dyspnea is the anterior insula, with stronger activations on the right than on the left (Fig. 55-1A and B). The anterior insula is activated by several other unpleasant sensations that provide information on primal biologic drives, such as pain, thirst, and hunger.29,50,51 Air hunger also activates the amygdala, which is implicated in the perception of fear and anxiety (Fig. 55-1C and D).
Figure 55-1
Functional brain images showing some important paralimbic activations during laboratory-induced air hunger (data from Evans et al29). Air hunger was induced during volume-control ventilation by lowering tidal volume (VT); red ovals in A and B indicate activations in the anterior insular cortex (IC). The sagittal section (A) is in the right hemisphere, 34 mm from the midline; the coronal section (B) is 16 mm forward of the anterior commissure. The anterior insula is also involved in hunger, thirst, and pain. Green ovals in C and D indicate activations in the amygdala. The sagittal section (C) is in the right hemisphere, 24 mm from the midline; the coronal section (D) is 4 mm forward of the anterior commissure. The amygdala is implicated in states of fear and anxiety. These images were obtained using blood oxygen level dependent functional magnetic resonance imaging. All activations shown were statistically significant after correction for multiple comparison.
There is a substantial amount of information on the interaction of blood-gas levels, level of minute ventilation, and air hunger during mechanical ventilation in normal subjects and in patients who are ventilator-dependent secondary to neuromuscular paralysis. In a study of sixteen healthy subjects ventilated at 10 L/min, end-tidal carbon dioxide tension (PETCO2) was raised by increasing inspired CO2. An increase of 10 torr produced a level of respiratory discomfort that subjects could not tolerate even for a few minutes (Fig. 55-2).15 This occurred despite a background of high oxygen and with minute ventilation () greater than 150% of the normal resting level. In practical terms, this implies that modest acute increases in partial pressure of carbon dioxide (PCO2) that may seem clinically unimportant can be a source of profound discomfort.
Figure 55-2
Air hunger response to an acute rise in end-tidal carbon dioxide tension (PETCO2) produced by inspired CO2 in normal healthy men and women (closed circles). The prevailing chronic level of PETCO2 is indicated by a triangle. Bars indicate standard error of the mean. Volume-control ventilation was delivered via a mouthpiece at 0.16 L · min−1 · kg−1. Subjects in these experiments were instructed to rate “discomfort due to your urge to breathe.” “Extreme” (Extr) on the rating scale was defined to the subjects as an intolerable level, and “moderate” (Mod) was defined as a level that could be tolerated for several minutes. (Data were replotted from Banzett et al.15)
An acute rise in PaCO2 evokes severely uncomfortable air hunger during volume-control ventilation in both healthy subjects and in alert patients ventilated for respiratory muscle paralysis.23,38,39 The sensations of air hunger and work/effort produced by mild hypercapnia in spontaneously breathing healthy subjects are increased by partial neuromuscular block, even at PETCO2 and levels similar to those present before the block.52,53 This may explain why exertional dyspnea occurs in patients with profound neuromuscular weakness.
How do responses to chronically altered blood gases compare to the acute stimulus–response relationships for air hunger detailed above (which were obtained in exposures of 10 minutes or less)? Common clinical experience leads to the hypothesis that there is adaptation to chronic changes in blood gases; for instance, the common observation that some patients with chronic obstructive pulmonary disease do not report dyspnea at resting PCO2 in excess of 50 torr, a level that is intolerable to normal subjects at comparable ventilation levels. A direct test of the hypothesis was obtained in mechanically ventilated patients who adapted to a gradual 15-torr rise in PaCO2 produced by slowly raising inspired PCO2 while holding ventilation and partial pressure of arterial oxygen (PaO2) constant.23 The air hunger response to acute hypercapnia was tested before and after the adaptation period. Within 2 to 3 days, the acute response to hypercapnia had also shifted by 15 torr, essentially complete adaptation (Fig. 55-3). The authors attributed this shift to neural adaptation, rather than acid–base compensation; thus, it may apply to other stimuli such as hypoxia.
Figure 55-3
Adaptation of air hunger response to prevailing chronic level of PETCO2 (triangles) in four ventilator-dependent patients. Response to acute hypercapnia (circles) is shown at baseline chronic level of PETCO2 (filled symbols; mean of 27 torr in these chronically overventilated patients), and after adaptation for several days to a mean PETCO2 of 41 torr (open symbols). Bars indicate standard error of the mean. The increase in chronic partial pressure of carbon dioxide (PCO2) was effected by adding inspired CO2 at the same minute ventilation (). (Data replotted from Bloch-Salisbury et al.23)
Subjects report little or no difference between the quality of air hunger evoked by hypoxia versus that evoked by hypercapnia.46 If PCO2 and ventilation are near normal, pronounced hypoxia is required to evoke air hunger; in healthy subjects an arterial partial pressure of oxygen (PO2) of 40 to 50 torr produces only mild air hunger if PaCO2 remains at eucapnic levels.46 The effects of hypoxia are enhanced by hypercapnia.
Mechanoreceptor input arising from breathing can dramatically reduce air hunger, as demonstrated by the classic experiment in which subjects held their breath until breakpoint and then breathed from a bag containing gas with alveolar concentrations of CO2 and O2, preventing improvement of arterial blood gases.16,54 Subjects reported immediate relief and could even continue with another breathhold. This phenomenon is manifested during mechanical ventilation as a decrease in dyspnea when tidal volume is increased, as was initially shown in polio patients.55 Subsequently, mechanoreceptor relief of air hunger has been shown in normal subjects.29,50,56 Studies of healthy subjects on volume-control ventilation, who have been initially made uncomfortable with mildly elevated PCO2, show that there is a decline in discomfort (air hunger) as is increased with constant blood-gas levels (the solid line in Fig. 55-4). In these subjects, zero discomfort can be achieved, and further increases have no effect, or produce a different form of discomfort, “too much pressure.” In normal subjects, the relationship between ventilation and air hunger is linear (A.P. Binks, personal communication). This relief is undiminished in C1–C2 quadriplegic patients who have no motor or sensory innervation of the rib cage and diaphragm, showing that pulmonary stretch receptors that send impulses via the vagus nerve, are able to produce the full response57 (dotted line in Fig. 55-4). It is not clear, however, whether chest wall receptors form a fully redundant pathway capable of providing relief. Tidal volume provided somewhat less relief in double-lung transplant patients than in normal subjects;56 however, even the diminished response present in these transplant patients may have been caused by reinnervation of the transplanted lung, because the patients were studied 1 to 9 years posttransplantation.58
Figure 55-4
Relief of air hunger by increased minute ventilation at constant PETCO2 in normal subjects (solid line, closed symbols) and patients with high cervical quadriplegia (dotted line, open symbols). In all cases, air hunger was generated by adding inspired CO2 to increase PETCO2 above the subjects’ baseline PETCO2. (Mean PETCO2 in normal subjects was 44 torr and in quadriplegic patients, 34 torr, owing to their lower baseline PCO2.) PETCO2 and respiratory frequency were held constant as ventilator tidal volume was varied. (Data for normal subjects combined from Harty et al56 and Banzett et al50; data for quadriplegic patients combined from Manning et al57 and Bloch-Salisbury et al.90)
The sense of breathing work or effort arises both from muscle receptors and from corollary discharge arising from cerebral motor cortex activation during volitional respiratory efforts.59 (Corollary discharge from medullary respiratory centers probably does not give rise to increased work or effort of breathing.) Although some studies of well-trained subjects have shown a distinction between effort and work, most normal subjects and patients do not distinguish the two; thus, we use work/effort to denote the sensation. Work/effort of breathing is perceived when the physical work of breathing is increased by loading the respiratory muscles with high or increased impedance, or when increased cortical motor drive is necessitated by respiratory muscle weakness.53,60–62
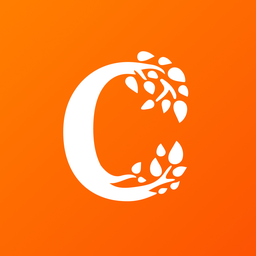
Full access? Get Clinical Tree
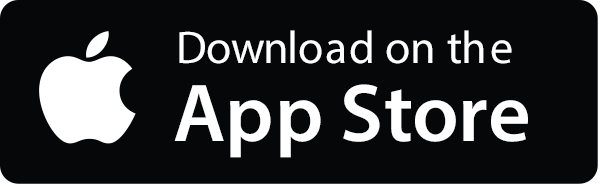
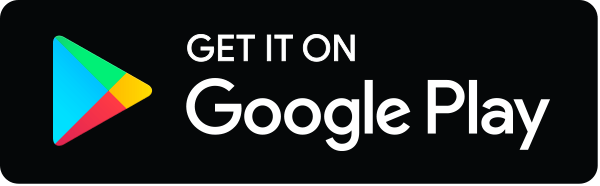
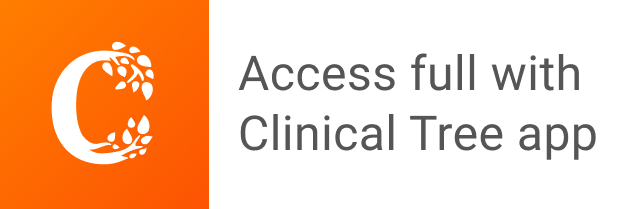