Acute Inhalation Injury
David J. Prezant
Dorsett D. Smith
Lawrence C. Mohr Jr
Overview
Chemicals with potential toxicity are regularly used and produced in a variety of industrial processes. If inhaled, many have the potential to cause asphyxiation or life-threatening acute lung injury. Although recent events have increased concern that toxic gases may be used as weapons of mass destruction, accidental exposures remain the greatest health threat [1]. Individuals may be exposed to the accidental release of toxic gases in the workplace [2] or in the general environment, including the home [1].
Smoke inhalation is another major cause of acute inhalation injury [3]. Thousands of individuals become smoke inhalation victims each year, having been exposed to toxic gases and airborne particulate matter from the burning of a variety of materials [4]. Smoke inhalation most commonly occurs as a result of industrial or residential fires, where large amounts of carbon monoxide, hydrogen cyanide (HCN), hydrogen chloride, acrolein, sulfur dioxide, phosgene, and other toxic, irritant gases are produced (Table 64.1). It remains the primary cause of death in approximately 80% of burn injury victims in the United States.
Toxic agents can be inhaled in several different physical states. A gas is a substance that, at standard temperature and pressure, has the ability for its molecules to diffuse freely and be distributed uniformly throughout any container. A gas in the atmosphere has the capability of infinite expansion. The density of a gas is expressed relative to air. The denser the gas, the heavier it is. Gases that are denser than air will typically gravitate to low areas. Cold gases are denser than the same gas at higher temperatures. A vapor is a substance in the gaseous state that normally exists as a liquid or solid and is formed when a substance is heated above its critical temperature, which is the temperature at which it cannot be liquefied regardless of the amount of pressure applied. A fog is a liquid aerosol formed by a condensation of a substance from a gaseous state to a liquid state. Dusts are fine particles of a solid organic or inorganic material that are small enough to be airborne, typically ranging from 0.1 to 25.0 μm in diameter. Fumes are extremely fine solid particles that are dispersed into the air by the combustion or melting of solid materials, particularly metals. Fumes usually consist of particles that range from 0.001 to 1.0 μm in diameter. Smoke consists of airborne particles resulting from the incomplete combustion of organic materials. These particles either contain or are coated with multiple chemical substances resulting from combustion and range in size from less than 0.3 μm to greater than 10 μm in diameter.
Table 64.1 Toxic Products of Combustion in Residential Fires | ||||||||||||||
---|---|---|---|---|---|---|---|---|---|---|---|---|---|---|
|
The nature of acute injury that an individual sustains after the inhalation of a toxic substance will depend on the chemical and physical properties of the inhaled toxicant, the pathophysiological mechanism by which the toxicant causes injury, the dose received, and whether prior pulmonary disease exists. This chapter will focus on the diagnosis and treatment of acute inhalation injury resulting from asphyxiant gases, toxic irritant gases, and smoke.
Asphyxiant Gases
Background
Asphyxiants are gases that cause tissue hypoxia. They are classified as either simple asphyxiants or chemical asphyxiants
based on their mechanism of toxicity. Simple asphyxiants displace or dilute oxygen in the ambient atmospheric air causing a decrease in the fraction of oxygen in inspired air (FIO2). Chemical asphyxiants, on the other hand, interfere with physiological processes associated with the uptake, transport, or utilization of oxygen. Simple asphyxiants include common gases such as carbon dioxide, natural gas, propane, methane, nitrogen, and acetylene. They may be lighter or heavier than air (Table 64.2). Simple asphyxiants that are lighter than air accumulate and displace oxygen in higher areas first, whereas those that are heavier than air accumulate and displace oxygen in low-lying areas first. Chemical asphyxiants can be further characterized as those that decrease oxygen-carrying capacity, such as carbon monoxide, and those that inhibit oxygen utilization by cells, such as HCN (Table 64.3). Medical problems related to the inhalation of the most common asphyxiants are discussed in the sections that follow.
based on their mechanism of toxicity. Simple asphyxiants displace or dilute oxygen in the ambient atmospheric air causing a decrease in the fraction of oxygen in inspired air (FIO2). Chemical asphyxiants, on the other hand, interfere with physiological processes associated with the uptake, transport, or utilization of oxygen. Simple asphyxiants include common gases such as carbon dioxide, natural gas, propane, methane, nitrogen, and acetylene. They may be lighter or heavier than air (Table 64.2). Simple asphyxiants that are lighter than air accumulate and displace oxygen in higher areas first, whereas those that are heavier than air accumulate and displace oxygen in low-lying areas first. Chemical asphyxiants can be further characterized as those that decrease oxygen-carrying capacity, such as carbon monoxide, and those that inhibit oxygen utilization by cells, such as HCN (Table 64.3). Medical problems related to the inhalation of the most common asphyxiants are discussed in the sections that follow.
Table 64.2 Simple Asphyxiants | ||||||||||||||
---|---|---|---|---|---|---|---|---|---|---|---|---|---|---|
|
Carbon Dioxide
Pathophysiology
Carbon dioxide (CO2) is the most common simple asphyxiant. It is produced by aerobic metabolism and is exhaled into the atmosphere by humans and other animals. It is also a byproduct of carbohydrate fermentation, the combustion of carbonaceous material, and the oxidation of coal contaminants in coal mines. It exists in the frozen form as dry ice. CO2 is heaver than air and reduces FIO2 simply by diluting and displacing oxygen in ambient air. Most deaths from CO2 asphyxiation result from the confinement of an individual in enclosed or poorly ventilated space. Such closed-space confinement prevents air with a normal FIO2 from entering while exhaled CO2 is accumulating and displacing oxygen inside. Simple asphyxiation from CO2 has also been reported from environmental exposures. In 1986, for example, simple asphyxiation caused approximately 1,700 deaths from a cloudy mist of CO2 and water droplets that rose suddenly from a lake in Cameroon [5]. Asphyxiation from CO2 has also been reported by off-gassing from dry ice in a confined space [6].
Table 64.3 Chemical Asphyxiants | |
---|---|
|
In general, once the ambient CO2 increases to the point where the FIO2 has decreased to 0.15, acute signs and symptoms of hypoxia begin to appear within minutes. These include dyspnea, tachypnea, tachycardia, confusion, incoordination, and dizziness. As the FIO2 decreases below 0.10, lethargy or coma may develop as a result of cerebral edema, and cardiopulmonary arrest may occur. Brain damage sustained as a result of extensive cerebral edema or prolonged hypoxia may be permanent in individuals with these conditions who are resuscitated and survive. It is unlikely that life can be sustained for more than several minutes with a FIO2 less than 0.06 [7].
Diagnosis and Management
CO2 asphyxiation should be considered in any patient who presents with clinical signs of hypoxia, is unconscious, or is found to be in cardiopulmonary arrest after removal from an enclosed space or another source of potential CO2 exposure. Clinical signs are nonspecific and related to the magnitude of hypoxia, as indicated earlier. Arterial blood gases, serum electrolytes, and measurement of the anion gap should be obtained. During and shortly after CO2 asphyxiation, arterial blood gas analysis would be expected to show decreased arterial oxygen tension (PaO2) and elevated carbon dioxide tension (PaCO2). However, both PaO2 and PaCO2 typically return to normal shortly after the patient is removed from the source of CO2 exposure. Once the patient breathes oxygenated air, CO2 is rapidly excreted by hyperventilation. Most patients will be acidotic at the time of presentation as a result of respiratory acidosis from CO2 retention and concurrent lactic acidosis from hypoxia. Lactic acidosis will cause an elevated anion gap. The respiratory acidosis typically resolves shortly after removal from the source of CO2 exposure. The lactic acidosis will resolve once tissue oxygenation returns to normal but usually takes longer to resolve than the respiratory acidosis. The hypoxia caused by CO2 asphyxiation can cause cardiac dysrhythmias and myocardial infarction, especially in individuals with underlying heart disease. Therefore, it is recommended that an electrocardiogram and serial cardiac biomarkers be obtained on all patients.
Removal from the source of exposure and administration of oxygen are the only specific therapies for CO2 asphyxiation. If the patient is alert, has spontaneous respirations, and has a patent airway, it is recommended that high-flow oxygen be administered by a nonrebreather mask. Endotracheal intubation will be required if adequate oxygenation cannot be achieved by the use of a face mask or the patient has suffered mental status changes or cardiopulmonary arrest. Additional supportive care, such as cardiopulmonary resuscitation, hemodynamic support, manual ventilation, and mechanical ventilation should be used as required by the patient’s overall condition. Cardiac dysrhythmias and myocardial infarction should be aggressively treated. Most victims of CO2 asphyxiation will recover completely if removed from the source of CO2 exposure prior to cardiopulmonary arrest and given medical treatment as soon as possible. Individuals who have experienced a prolonged period of hypoxia, however, may have irreversible brain damage and chronic neurological sequelae if they are successfully resuscitated.
Carbon Monoxide
Pathophysiology
Carbon monoxide (CO) is a colorless, odorless, tasteless, nonirritating gas. It is the most common chemical asphyxiant and the
second most common atmospheric pollutant after carbon dioxide. CO is produced in a variety of ways, including incomplete combustion from fires, faulty heating systems, internal combustion engines (including gas-powered generators placed in poorly ventilated areas during electrical failures), wood stoves, charcoal grills, volcanic eruptions, and a variety of industrial processes. In vivo hepatic production of CO occurs in poisoning from methylene chloride that is commonly found in paint thinners and is easily absorbed through the skin.
second most common atmospheric pollutant after carbon dioxide. CO is produced in a variety of ways, including incomplete combustion from fires, faulty heating systems, internal combustion engines (including gas-powered generators placed in poorly ventilated areas during electrical failures), wood stoves, charcoal grills, volcanic eruptions, and a variety of industrial processes. In vivo hepatic production of CO occurs in poisoning from methylene chloride that is commonly found in paint thinners and is easily absorbed through the skin.
More than 5,000 deaths are attributed to CO poisoning in the United States each year [8]. Most are intentional from exposures to motor vehicle exhaust. The minority are accidental and due to fires or the use of poorly ventilated generators following storms, blackouts, or other disasters [9]. CO poisoning is responsible for 80% of fatalities related to smoke inhalation [10,11]. Twenty-five percent of fatalities from CO poisoning occur in persons with underlying cardiopulmonary disease [11,12].
Upon inhalation, CO easily diffuses across alveolar-capillary membranes in the lung and is rapidly taken up by erythrocytes in the pulmonary capillary blood. It binds to the iron moiety of hemoglobin with an affinity that is approximately 240 times greater than the affinity of hemoglobin for oxygen. Thus, CO competes with oxygen for hemoglobin binding sites and, as a result of its greater affinity, displaces oxygen from hemoglobin. The binding of CO to the iron moiety also creates an allosteric change in the hemoglobin molecule that inhibits the off-loading of oxygen in the peripheral tissues and causes a shift of the oxyhemoglobin dissociation curve to the left. CO also interferes with intracellular oxygen utilization by inactivating intracellular respiratory enzymes, such as cytochrome oxidase [13]. Thus, the cumulative effect on peripheral oxygen delivery and utilization is greater than that expected from decreased oxygen transport alone [14]. Reoxygenation injury of the brain has also been described [15]. One mechanism for reoxygenation injury appears to be lipid peroxidation of the brain by xanthine oxidase that is generated by peroxidases and reactive oxygen species produced by activated neutrophils that become sequestered in the microvasculature of the brain following, but not during, CO poisoning [16]. In summary, CO toxicity involves four pathophysiological mechanisms: (a) a decrease in the oxygen-carrying capacity of blood; (b) decreased oxygen delivery to peripheral tissues as a result of the left shift in the oxyhemoglobin dissociation curve; (c) mitochondrial dysfunction and impairment of cellular respiration by inhibition of cytochrome oxidase activity; and (d) lipid peroxidation of the brain during reoxygenation. It has been suggested that an immunological response to myelin basic protein may also be involved in the delayed neurological dysfunction that is seen in over half of those with serious CO poisoning between 3 days and 4 weeks after exposure [17].
The clinical presentation of individuals with CO poisoning is highly variable with nonspecific symptoms and signs that are loosely correlated to carboxyhemoglobin levels (Table 64.4). Early symptoms of CO poisoning include headache, dizziness, sore throat, nausea, shortness of breath, and fatigue. These symptoms can mimic those of a nonspecific viral syndrome, especially when an entire family is affected from CO exposure related to a faulty home heating system during the winter months. Impaired ability to concentrate occurs in more than half of affected individuals, and 6% have been reported to experience loss of consciousness. The severity of symptoms appears to correlate better with duration of exposure than with carboxyhemoglobin levels [18]. The brain and heart are very sensitive to CO intoxication, and both neurologic and cardiovascular impairment predominate with prolonged exposures. Mental status changes, and seizures, loss of consciousness, tachypnea, tachycardia, cardiac dysrhythmias, hypotension, and myocardial ischemia are likely to occur when the carboxyhemoglobin concentration exceeds 20%. Loss of consciousness may then occur rapidly and without warning. Cardiovascular disorders may occur at lower concentrations in subjects with preexisting cardiopulmonary diseases. Evidence of myocardial ischemia has been observed in one third of individuals with moderate-to-severe CO intoxication, and it has recently been reported that myocardial injury, as determined by elevation of serial cardiac biomarkers, is an independent predictor of mortality from CO poisoning [12,19,20]. Metabolic acidosis, as a result of increased lactate production from anaerobic metabolism, is a common consequence of tissue hypoxia. Rhabdomyolysis can occur as a consequence of impaired aerobic metabolism in skeletal muscle cells. Renal failure can develop as a consequence of rhabdomyolysis, but this occurs infrequently [21]. Carbon monoxide poisoning is almost always fatal when the carboxyhemoglobin concentration exceeds 60% [10,22].
Table 64.4 Carbon Monoxide Toxicity | ||||||||||||||||
---|---|---|---|---|---|---|---|---|---|---|---|---|---|---|---|---|
|
Fetal hemoglobin has a much greater affinity for CO than adult hemoglobin. Therefore, during pregnancy, the fetus may be more susceptible to CO poisoning than the mother. Once the mother is removed from the source of CO, clearance of carboxyhemoglobin may take four to five times longer in the fetus than it did in the mother [23]. Thus, the effective duration of CO exposure is considerably longer for the fetus than it is for the mother. It has been reported that severe CO toxicity in pregnant women can produce ischemic brain damage to the fetus and increase the risk of stillbirth [24,25].
Carbon monoxide poisoning can result in a delayed neuropsychiatric syndrome that may present at any time between 3 days and 4 months after apparent recovery from acute effects [10,26]. The syndrome has been reported to occur in 10% to 30% of individuals who survive CO poisoning. Symptoms include cognitive impairment, personality changes, parkinsonism, incontinence, focal neurological deficits, dementia, and psychosis. There is poor correlation between the development of the delayed neuropsychiatric syndrome and carboxyhemoglobin levels. Loss of consciousness during the acute illness phase, carboxyhemoglobin 25% or more, duration of exposure, and age appear to be significant risk factors (18). Brain imaging studies have shown that the areas most affected are the globus pallidus and deep white matter [10]. The exact mechanism for the development of this syndrome is unclear, but it is thought to be associated with reoxygenation brain injury, as discussed earlier. Most affected individuals recover within 1 year, although some may have chronic, long-term neurological or psychiatric impairment [10].
Diagnosis and Management
Because CO poisoning can present with a variety of nonspecific signs and symptoms, a high index of suspicion is needed to make the diagnosis. Cherry-red lips, cyanosis, and retinal hemorrhages have been reported in some cases of high-dose CO poisoning, but these signs occur infrequently and diagnosis depends on clinical history substantiated by increased levels of carboxyhemoglobin in arterial or venous blood [10]. Carboxyhemoglobin is most accurately measured by cooximetry because routine pulse oximetry cannot distinguish between carboxyhemoglobin and oxyhemoglobin. PaO2 is also of little value, since in the absence of coexistent lung injury it is normal. This is due to the fact that a CO partial pressure of only 1 mm Hg in arterial blood can saturate more than 50% of hemoglobin without affecting gas exchange or the amount of dissolved oxygen.
Recently, noninvasive cooximetry has become commercially available. Studies show that it has a high degree of specificity but poor sensitivity [27,28]. Using a cutoff of 15% carboxyhemoglobin, noninvasive cooximetry had a poor sensitivity of 48% (correctly identified only 11 of 23 patients with elevated levels) but an excellent specificity of 99% (correctly identify 96 of 97 patients with levels below 15%) [28]. Until further studies are done, this would suggest that its primary value is ruling out the diagnosis when there are no symptoms. It is probably most useful in environments where it is difficult or not possible to obtain blood measurement such as by Emergency Medical Service (EMS) units in the prehospital environment [29].
The evaluation of patients with CO poisoning should also include a thorough examination for evidence of thermal injury to the skin or airways. If CO poisoning is the result of a suicide attempt, a drug screen and serum ethanol, salicylate, and acetaminophen levels should be obtained. Another advantage of measuring the arterial carboxyhemoglobin level is that it also allows for simultaneous measurement of arterial pH. The pH can be used in conjunction with the anion gap and the serum lactate level to assess the degree of metabolic acidosis which when elevated is an independent predictor of poor prognosis [10]. PaCO2 is only helpful in assessing the ventilatory response to hypoxia and ventilatory compensation for lactic acidosis and should be obtained when mental status is abnormal or there is a prior history of chronic pulmonary disease. The serum creatine kinase level will be elevated if rhabdomyolysis has occurred. An electrocardiogram and serial cardiac biomarkers should be obtained in all patients to evaluate the possibility of myocardial ischemia or infarction. Because CO lowers the threshold for the development of ventricular dysrhythmias, patients should be carefully monitored until they are discharged from the emergency department or hospital [30]. The chest radiograph is usually normal, but signs of noncardiogenic pulmonary edema can rarely be seen in cases of severe CO poisoning [22], especially if there is coexistent smoke inhalation. Computed tomography (CT) of the head is useful if there is a need to rule out other causes of neurological impairment in this acute setting.
The initial treatment of CO poisoning is prompt removal from the source of exposure and administration of 100% oxygen via a nonrebreather mask to reduce the half-life of carboxyhemoglobin from 4 to 6 hours to 40 to 80 minutes [10,31]. Patients who are unconscious or have cardiopulmonary compromise should be intubated and receive 100% oxygen by mechanical ventilation and hyperbaric oxygen therapy (HBOT) be considered (see later). Oxygen should be administered until the carboxyhemoglobin level returns to normal. Pregnant women typically require oxygen for a longer period of time, because it takes longer for CO to be excreted from the fetus as a result of the greater affinity of fetal hemoglobin for CO [23].
Most patients with mild-to-moderate CO poisoning can be treated in the emergency department and discharged after the carboxyhemoglobin level has returned to normal and all abnormal signs and symptoms have resolved. Patients with severe CO poisoning, coexistent smoke inhalation, serious underlying diseases, neurologic or cardiopulmonary instability, or whose poisoning was an intentional suicide attempt should be admitted to the hospital for treatment and close observation.
HBOT has been used to treat patients with either extreme levels of CO poisoning (≥ 25% carboxyhemoglobin) or end-organ sensitivity to CO at elevated but lower levels. Examples of this might include neurologic abnormalities or hemodynamic instability that was felt to be caused by CO poisoning. HBOT is performed by placing the patient in a chamber that is highly pressurized with 100% oxygen. HBOT produces a large increase in the amount of dissolved oxygen in blood that in turn greatly increases the partial pressure of oxygen in the blood. The half-life of carboxyhemoglobin decreases as the partial pressure of oxygen in the blood increases. HBOT with 100% oxygen at a pressure of 2.5 to 3.0 atmosphere will reduce the half-life of carboxyhemoglobin from 4 to 6 hours to approximately 20 minutes [10,22,31].
Several animal studies suggest that HBOT may attenuate the development of delayed neuropsychiatric symptoms following CO exposure [32]. Although, the efficacy of HBOT for preventing the development of the delayed neuropsychiatric syndrome in humans following CO poisoning has not been conclusively established [33], many experts argue for its use when levels exceed 20% to 25% [33,34]. HBOT will, however, hasten the resolution of symptoms and when available is currently recommended for patients with CO poisoning meeting any of the following criteria: any period of unconsciousness, coma, or persistent neurologic abnormalities; carboxyhemoglobin level of 25% or more; metabolic lactic acidosis; or cardiac dysrhythmias [10,12,18,26,35,36,37]. If myocardial ischemia is present, most experts believe cardiac catheterization with stenting of the blocked vessel to be the urgently required procedure. In a pregnant patient, fetal distress even at lower percentage of carboxyhemoglobin elevations would prompt consideration for HBOT if available.
The clearance of CO can also be accelerated by use of normocapnic hyperoxic hyperpnea. In this technique, the patient breathes a hyperoxic gas mixture that contains an FIO2 of 95.2% to 95.5% and a small amount of CO2, in the range of 4.5% to 4.8%, through a nonrebreathing circuit. The resulting increase in minute ventilation increases the partial pressure gradient for oxygen and CO between pulmonary capillary blood and alveolar gas but does not increase the partial pressure gradient for CO2. In a clinical study, normocapnic hyperoxic hyperpnea reduced the half-life of carboxyhemoglobin to 31 minutes in comparison with 78 minutes in individuals treated with 100% oxygen at normal minute ventilation [38]. CO-poisoned patients in hospitals without access to hyperbaric chambers might benefit from this technique.
In addition to controversy concerning which patients with CO intoxication might benefit most from HBOT, there also exists controversy surrounding the need to treat for HCN toxicity (see later) in patients suffering severe CO poisoning from smoke inhalation. The likelihood for cyanide toxicity in smoke inhalation victims increases with increasing carboxyhemoglobin levels and increasing acidosis [39].
Hydrogen Cyanide
Pathophysiology
Hydrogen cyanide (HCN) is a chemical asphyxiant produced by the combustion of nitrogen-containing polymers during fires [39,40,41]. It is also part of jewelry making and various manufacturing processes (metal plating) and in the reclamation of
silver from photographic and radiographic film. It has the potential to be used as a chemical agent in terrorist attacks [42]. It is a colorless, volatile liquid at room temperature but readily vaporizes into a gas. The gaseous form of HCN easily diffuses across the alveolar membrane after inhalation. Inhaled HCN is lethal in high doses, and its inhalation during a fire can contribute to the mortality of smoke inhalation victims [39,40,41]. The inhalation of lethal doses of HCN may also occur following accidental releases at industrial facilities or from its use in a terrorist attack.
silver from photographic and radiographic film. It has the potential to be used as a chemical agent in terrorist attacks [42]. It is a colorless, volatile liquid at room temperature but readily vaporizes into a gas. The gaseous form of HCN easily diffuses across the alveolar membrane after inhalation. Inhaled HCN is lethal in high doses, and its inhalation during a fire can contribute to the mortality of smoke inhalation victims [39,40,41]. The inhalation of lethal doses of HCN may also occur following accidental releases at industrial facilities or from its use in a terrorist attack.
After inhalation, HCN is rapidly distributed to tissues throughout the body. At the cellular level, HCN molecules bind to iron-containing sites on cytochrome a3 in mitochondria that inhibits the enzyme’s activity toxicity and decreases the cellular utilization of oxygen [39,42]. Cytochrome a3 is a key enzyme in the cytochrome oxidase system that is important for carrying out and sustaining aerobic metabolism within cells. Inhibition of cytochrome a3 by HCN will stop cellular respiration and oxidative phosphorylation, forcing affected cells into anaerobic metabolism. The binding of HCN to cytochrome a3, and the resulting inhibition of cellular respiration, can occur very rapidly after HCN is inhaled, with clinical signs and symptoms typically occurring within 15 seconds after inhalation.
The clinical effects of HCN intoxication are directly related to its ability to stop cellular respiration. They are nonspecific and identical to the signs and symptoms typically seen during hypoxia. Hyperpnea, dyspnea, tachycardia, agitation, anxiety, dizziness, headache, confusion, nausea, muscle weakness, and trembling are common. Lactic acidosis occurs as a result of anaerobic metabolism and may be severe. Hypotension, flushing, seizures, and Parkinson-like symptoms may occur in cases of severe intoxication. Coma, apnea, and cardiac dysrhythmias are poor prognostic signs unless prompt treatment is given [42,43].
Diagnosis and Management
The diagnosis of HCN poisoning requires a high index of suspicion. It should be suspected in every individual with any of the above signs or symptoms for which there is no other obvious cause. It should routinely be suspected in smoke inhalation victims, victims of industrial accidents in which cyanide could have been released, and victims of terrorist attacks. Blood and urine cyanide concentrations can be obtained, but the results of these tests are usually confirmatory and because these tests are not routinely performed in most laboratories, results can only be used to confirm the diagnosis. Treatment for this potentially life-threatening poisoning must be initiated based on diagnostic suspicion alone.
There are several important clues that can be helpful in making a clinical diagnosis of HCN intoxication. In smoke inhalation victims, HCN toxicity should be suspected whenever CO intoxication occurs, and in fact, the likelihood increases with increasing carboxyhemoglobin levels [39]. Regardless of the etiology of HCN exposure, metabolic acidosis with an increased anion gap and an elevated serum lactate concentration should typically be present. Arterial and venous blood gases can provide potentially useful information. Arterial oxygen tension is usually above 90 mm Hg, whereas venous oxygen tension may be significantly elevated above the normal range of 35 to 45 mm Hg because of poor cellular extraction and utilization of oxygen. Similarly, arterial oxygen saturation is typically in the normal range of 95% to 100%, whereas the oxygen saturation of mixed venous blood may be in the vicinity of 85% or greater. Thus, the mixed venous oxygen saturation may be significantly higher than the normal range of 60% to 80%. This so called arteriolarization of venous blood can be a useful clue in considering the diagnosis of HCN intoxication [44].
Because HCN poisoning can rapidly progress, treatment must begin as soon as possible in patient presenting with seizures, coma, hypotension, or cardiac arrest in whom HCN toxicity is suspected [45,46]. The United States Food and Drug Administration has approved two forms of therapy for cyanide toxicity. The newest is the Cyanokit antidote consisting of Hydroxocobalamin, a precursor to vitamin B12. It is a relatively benign substance with minimal side effects and rapid onset of action. For these reasons, it may be a superior antidote to the older more commonly available cyanide antidote kit (CAK) consisting of sodium nitrite and sodium thiosulfate [47,48]. Hydroxocobalamin has no adverse effect on the oxygen-carrying capacity of the red blood cells and no negative impact on the patient’s blood pressure—significant benefits when treating victims of smoke inhalation. The mechanism of action is surprisingly simple: Hydroxocobalamin binds to cyanide forming vitamin B12 (cyanocobalamin), a nontoxic compound excreted in the urine. Patients tolerate the drug without hypotension or allergic reactions. Quickly passing side effects include reddish color to the skin, urine, and mucous membranes, which may interfere with some colorimetric laboratory tests (i.e., blood glucose, iron levels, creatinine, total hemoglobin concentration, carboxyhemoglobin, oxyhemoglobin, methemoglobin) [49,50]. Victims presenting with seizures, hypotension, or a coma in a setting consistent with cyanide toxicity should be considered candidates for empiric administration of Hydroxocobalamin 5 gm IV over 15 minutes through two intravenous or intraosseous lines. Consideration should be given to obtaining a blood sample for subsequent analysis for HCN and for baseline laboratory tests that could be interfered with by the presence of hydroxocobalamin.
Sodium nitrite and sodium thiosulfate can also be used for the treatment of HCN poisoning. These antidotes are found in the CAK, along with ampules of amyl nitrite inhalant. Sodium nitrite generates methemoglobin by changing the normal ferrous state of iron in the heme molecule of hemoglobin (Fe+2) to the ferric state (Fe+3). The ferric heme molecules in methemoglobin have a high affinity for HCN. Thus, HCN molecules preferentially bind to the methemoglobin generated by sodium nitrate, which in turn prevents HCN from entering cells and inhibiting cellular respiration. The adult dose of sodium nitrite is 300 mg in 10 mL of diluent (30 mg per mL) administered intravenously over 2 to 4 minutes and the pediatric dose is 0.33 mL per kg of a 3% solution, intravenously over 2 to 4 minutes, not to exceed 10 mL [42,43]. Following the administration of sodium nitrite, sodium thiosulfate should be administered intravenously. Sodium thiosulfate acts as a substrate for rhodanese, a detoxifying enzyme found in the liver. In the presence of sodium thiosulfate, rhodanese catalyzes the conversion of HCN cyanide to thiocyanate that is then excreted in the urine. The adult dose is 12.5 g of sodium thiosulfate in 50 mL of diluent (25% solution), administered intravenously at a rate of 3 to 5 mL per minute. The pediatric dose of sodium thiosulfate is 412.5 mg per kg (1.65 mL per kg) of a 25% solution, given intravenously at a rate of 3 to 5 mL per minute [42,44].
The inhalation of amyl nitrite from ampules can be used as a temporizing measure until venous access for the administration of sodium nitrite and sodium thiosulfate is obtained. The inhalation of amyl nitrite should never be considered a substitute for the administration of intravenous sodium nitrite and sodium thiosulfate. In fact, amyl nitrite can itself be associated with serious reactions such as hypotension, syncope, methemoglobinemia, and hemolysis in G6PD-deficient patients. These effects are more pronounced in children, the elderly, and in patients with cardiopulmonary diseases. Dose regimen is difficult to control and could even result in exposure of the healthcare provider to amyl nitrite’s adverse effects. For these reasons, administration of amyl nitrite may be unwarranted, especially since hydroxocobalamin is now available [51].
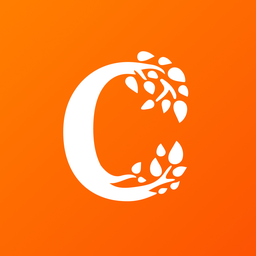
Full access? Get Clinical Tree
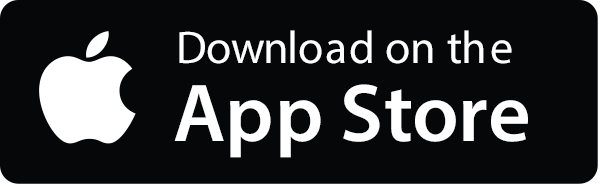
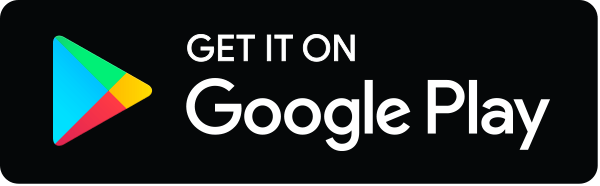
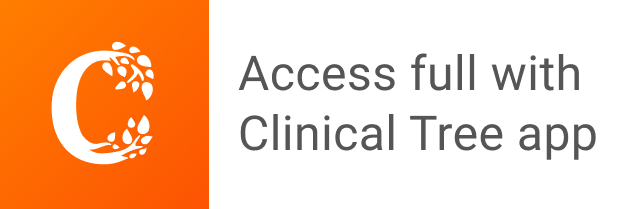