Acute Infectious Pneumonia
Veronica Brito
Michael S. Niederman
Pneumonia is a common community- and hospital-acquired infection that is managed in the intensive care unit (ICU) when it leads to acute respiratory failure or septic shock and complicates the course of an otherwise serious illness. Modern medical technology has not been able to eliminate this infection. Rather, it has promoted its emergence by the application of novel, life-sustaining therapies that lead to specific at-risk populations who have impairments in respiratory tract host defenses. This chapter reviews the scope of the problem in seriously ill patients.
Pneumonia occurs in up to 6 million outpatients annually (community-acquired pneumonia, CAP), with up to 1 million requiring hospitalization [1]. Pneumonia also develops in the hospital (nosocomial pneumonia or hospital-acquired pneumonia [HAP]), particularly in those patients with underlying serious illnesses, at the rate of approximately five to ten cases per one thousand hospital admissions [2]. In the hospital, the incidence of pneumonia is directly related to the degree of underlying systemic illness in a given patient, with the incidence being higher in medical than in surgical patients, and in those requiring prolonged mechanical ventilation than in those managed by short-term ventilatory support [2]. Recently, the distinction between CAP and HAP has become blurred, because patients with chronic illness often live in complex environments out of the hospital (nursing homes), or patients are repeatedly admitted to the hospital, or they receive treatments in healthcare settings such as dialysis centers. These individuals come in contact with the healthcare environment, even when they are not hospitalized, and can become infected with hospital-associated drug-resistant pathogens, and when they develop pneumonia, it is termed healthcare-associated pneumonia (HCAP) [2].
Certain patient populations are at increased risk for pneumonia, primarily as a result of disease-associated impairments in lung host defenses. These include the elderly and those with cardiac disease, alcoholism, chronic obstructive pulmonary disease (COPD), congestive heart failure (CHF), malnutrition, head injury, cystic fibrosis, bronchiectasis, malignancy, splenic dysfunction, renal failure, liver failure, diabetes mellitus, and any immunosuppressive illness or therapy [2,3]. In addition, hospitalized patients often receive therapeutic interventions that predispose them to pneumonia, including antibiotic therapy, enteral feeding, endotracheal intubation, tracheostomy, and the use of certain medications (such as corticosteroids, aspirin, digitalis, morphine, and pentobarbital) [3].
The mortality implications of pneumonia (along with influenza) rank it as the eighth leading cause of death in the United States, the sixth leading cause of death in those older than 65 years, and the number one cause of death from infectious diseases [4]. Although CAP can vary from a mild to a severe illness, those who enter the ICU with this infection have a mortality rate that can vary from 20% to greater than 50% [5]. Older studies questioned whether use of the ICU was even beneficial for severe CAP, but that was at a time when the ICU was only used when the disease was far advanced. In more recent studies, an effort has been made to identify patients with severe CAP at the earliest possible time point, and thus while as many as 90% of ICU admitted CAP patients in older studies were intubated and mechanically ventilated, more recently, only about 60% to 70% of CAP patients in the ICU receive this intervention. This means that the indications for ICU admission and the definitions of severe CAP are changing, and with good reason, since the later in the hospital course that the ICU is used, the higher the mortality [6]. Recently, Woodhead et al. [6] found that CAP accounted for 5.9% of all ICU admissions, but that early admission (within 2 days of hospitalization) appeared to be preferable and was associated with a lower mortality (46.3%) than late admission (> 7 days in the hospital, 50.4% mortality). Thus, the mortality associated with severe CAP is a reflection of how accurately the ICU is used, what organisms are causing the infection, what complications develop in the hospital, and how effective is the initial empiric therapy [7] (Table 68.1).
In data from the National Nosocomial Pneumonia Infection Surveillance System, pneumonia is the most common, ICU-acquired infection, with 86% of episodes being associated with mechanical ventilation [8]. Patients usually develop HAP because of an underlying chronic illness, and thus the question arises, if they die, whether their death was due to the pneumonia itself or a result of the underlying, predisposing illness. This issue of “attributable mortality” has been studied, and as many as 60% of those who die do so as a direct result of their pneumonia [9]. Not all studies report attributable mortality, particularly those involving surgical and trauma patients, a group that seems to acquire pneumonia commonly but usually without a major direct effect on mortality [10]. In those with acute respiratory distress syndrome (ARDS), the mortality rate of pneumonia has been reported to be high, with only 12% of patients with pneumonia surviving in contrast to 67% survival in the absence of infection [11]; however, more recent data report lower death rates from pneumonia in patient with ARDS.
Bacteriology is another important factor adding to mortality in HAP, with Kollef et al. [12] reporting a high attributable mortality for late-onset ventilator-associated pneumonia (VAP) caused by potentially drug-resistant organisms such as Pseudomonas aeruginosa, Acinetobacter spp, and Stenotrophomonas maltophilia. Rello et al. [13] matched patients with VAP caused by methicillin-resistant Staphylococcus aureus (MRSA) with controls having caused by other organisms. They found that the mortality for MRSA VAP was 48%, compared with 25% for control patients (p < 0.01). Heyland et al. [14] compared 177 patients with VAP to a matched control group of critically ill ventilated patients without pneumonia and found that patients with pneumonia had a longer duration of mechanical ventilation, longer stay, and a trend toward increased mortality, particularly with the use of initially inappropriate empiric antibiotic therapy. Thus, similar to the data with severe CAP, mortality in HAP is also affected by patient characteristics, bacteriology, and the accuracy of therapy. In studies of HCAP, when patients are admitted to the ICU, mortality can also be high, and it is increased if patients do not receive appropriate initial antibiotic therapy [15].
Table 68.1 Risk Factors for Pneumonia Mortality in Patients with Cap | |
---|---|
|
Types of Pneumonia Encountered in the Intensive Care Unit
Serious pneumonia occurs when a potential pathogen overwhelms a patient’s host defenses, and then, because of either overwhelming infectious challenge or an excessive inflammatory response to infection, the patient develops respiratory failure or septic shock. Certain pathogens are so virulent that they can even overcome an intact, and normal, host defense system, as is the case with epidemic viral illness. Normal host defenses can also be overcome if the inoculum of the pathogen is large (as with massive aspiration), but smaller inocula can be pathogenic if disease-associated factors interfere with immune function. Certain patients seem to become ill because of an excessive inflammatory response to a localized infection, and genetic polymorphisms in the immune response are being identified to explain this phenomenon.
Community-Acquired Pneumonias Leading to Intensive Care Unit Admission
Although less than 20% of all patients with CAP require hospitalization, those patients ill enough to enter the hospital may have a substantial mortality rate. As classically described by Austrian and Gold [16], for certain patients with advanced illness, even penicillin therapy could not eliminate the mortality of pneumococcal pneumonia, because the disease process was too advanced at the time of presentation.
When CAP leads to ARDS, a complication that occurs in less than 5% of cases, the mortality rate can exceed 70% [17]. For a general ICU population, the mortality rate of CAP, reported in a meta-analysis of 788 patients, was just more than 35%, and other series have reported even lower rates [18]. Pneumonia caused by bacteria, viruses, fungi, and protozoa can occasionally be severe enough to prompt admission to the ICU. Pathogens that have been described as causing severe CAP include Streptococcus pneumoniae (pneumococcus), Legionella pneumophila, Haemophilus influenzae, enteric Gram-negative bacteria, S. aureus (including community-acquired methicillin-resistant strains), Mycoplasma pneumoniae, Pneumocystis jiroveci, Mycobacterium tuberculosis, Chlamydophila pneumoniae, endemic fungi (blastomycosis, histoplasmosis) influenza virus, respiratory syncytial virus, varicella, severe acute respiratory syndrome virus (SARS, caused by a coronavirus), and the bacteria associated with aspiration pneumonia [4].
Definition of Severe CAP and Prognostic Factors/Scoring Systems
Although “severe” CAP does not have a uniform definition, the term has been used to refer to patients with CAP who require ICU care, although, recently, some investigators have focused on defining patients with CAP who need invasive respiratory or vasopressor support (IRVS), independently of site of admission [4,5]. Torres et al. [19] estimated that CAP accounted for 10% of all admissions to an ICU over a 4-year period, and that these patients were admitted directly to the ICU 42% of the time, after admission to another ward 37% of the time, and in transfer from another hospital 21% of the time.
There are some patients in whom pneumonia is such a virulent infection that survival may have already been determined when they reach the ICU because the patient is already “too far gone.” Some older studies questioned whether treating CAP patients in the ICU could even impact mortality, since as many as 75% of patients with pneumococcal pneumonia managed in the ICU died. However, in older series, most of these patients were mechanically ventilated when admitted to the ICU so that the ICU may have been used very late in the course of illness. In more recent studies, only about 60% to 70% of patients with CAP in the ICU receive mechanical ventilation [6]. In these series of severe CAP, the mortality rates have varied from 21% to 54%, with the lower mortality rates being found when not all patients were mechanically ventilated, and the higher mortality rates being seen when nearly 90% were being ventilated [5]. These findings suggest that there is value in defining the need for ICU care at the earliest possible time point, and not reserving the ICU for extreme circumstances such as overt respiratory failure and shock.
Poor prognostic factors in CAP are as follows: multilobar pneumonia, respiratory rate greater than 30 breaths per minute, severe hypoxemia, abnormal liver function, low serum albumin, signs of clinical sepsis, and delayed or inappropriate antibiotic therapy [4,18]. While sepsis increases CAP mortality, bacteremia by itself is not a mortality risk. In a recent study [20], bacteremia was not an independent mortality risk or a predictor of delayed clinical response, after controlling for other variables such as age, comorbidities, and abnormal vital signs at presentation.
Over the past decade, a number of studies have examined prognostic scoring systems for patients with CAP. In general, there are two widely used approaches, the Pneumonia Severity Index (PSI) and the British Thoracic Society approach (CURB-65). Each uses a point scoring system to predict a patient’s mortality risk, with the CURB-65 being simpler and more focused on acute illness parameters, whereas the PSI is a more complex system that incorporates measurements of both chronic and acute disease factors [5]). While both tools predict mortality risk, neither is a direct measure of severity of illness. For example, as many as 37% of those admitted to the ICU in one study [21] were in PSI classes I–III, pointing out that even those with a low risk for death (which PSI can measure) may benefit from aggressive intensive care support [21]. Conversely, patients in
higher PSI classes do not always need ICU care if they fall into these high mortality risk groups because of advanced age and comorbid illness in the absence of physiologic findings of severe pneumonia.
higher PSI classes do not always need ICU care if they fall into these high mortality risk groups because of advanced age and comorbid illness in the absence of physiologic findings of severe pneumonia.
In one recent study [22], both tools were applied to the same patients, and each was similarly accurate for identifying low-risk patients. However, the CURB-65 was more discriminating in predicting mortality risk for patients with more severe illness. This approach gives one point for each of five abnormalities: confusion, elevated blood urea nitrogen (BUN) (> 19.6 mg per dL), respiratory rate 30 per minute or more, low blood pressure (BP) (either systolic ≤ 90 mm Hg or diastolic ≤ 60 mm Hg), and whether the patient is at least 65 years old. If three of these five criteria are present, the predicted mortality rate is greater than 20%, and these patients are generally considered for ICU admission [23]. A similar approach has been developed by the Japanese Respiratory Society, the A-DROP scoring system, that assesses Age (male ≥ 70 years, female ≥ 75 years); Dehydration (BUN ≥ 210 mg per L); Respiratory failure (SaO(2) ≤ 90% or PaO(2) ≤ 60 mm Hg); Orientation disturbance (confusion); and low blood Pressure (systolic BP ≤ 90 mm Hg) [24].
Another scoring system, developed by España et al., based on data from 1,057 patients in Spain, suggested that the need for ICU admission could be defined by the presence of one of two major criteria (arterial pH < 7.39 or a systolic BP < 90 mm Hg), or the presence of two of six minor criteria, which included confusion, BUN greater than 30 mg per dL, respiratory rate greater than 30 per minute, PaO2/FiO2 ratio less than 250, multilobar infiltrates, and age 80 years or older. This approach gave different point values to each abnormality, and when severity criteria were met, the tool was 92% sensitive for identifying those with severe CAP and was more accurate than the PSI or the CURB-65 [25]. Another tool, the SMART-COP approach [26], was developed in Australia to predict the need for IRVS using eight clinical features, in which the acronym referred to systolic BP less than 90 mm Hg, multilobar infiltrates, albumin less than 3.5, respiratory rate elevation (> 25 for those younger than 50 years, and > 30 for those older than 50 years), tachycardia (> 125 per minute), confusion, low oxygen (< 70 mm Hg if younger than 50 years or < 60 mm Hg if older than 50 years), and arterial pH less than 7.35. The abnormalities in the systolic BP, oxygenation, and arterial pH each received 2 points, whereas the five other criteria received 1 point each, and with this system, the need for IRVS was predicted by a SMART-COP score of at least 3 points. Using this cutoff, the sensitivity for the need for IRVS was 92.3% and the specificity of 62.3%, with a positive and negative predictive value of 22% and 98.6%, respectively. Both PSI and CURB-65 did not perform as well overall.
The adverse prognostic factors discussed above are particularly applicable to the elderly, and those with nursing home–acquired pneumonia often have a higher mortality rate than those with simple CAP [5]. One factor that may explain this finding is that older patients often have atypical clinical presentations of pneumonia, which may lead to their being diagnosed at a later, more advanced stage of illness, resulting in an increased risk of death [5]. Older patients from nursing homes presenting with pneumonia are now included in a separate category, called HCAP.
Recently, the accuracy of prognostic scoring systems has been enhanced by biomarker measurements. Salluh et al. [27] and others have found that baseline cortisol levels showed a good correlation with CAP outcome. The most data have focused on procalcitonin (PCT), a “hormokine” that has increased plasma concentrations in the presence of severe bacterial infections, but not in viral illness. Masia et al. [28] found that PCT levels correlated well with the PSI score and the development of complications such as empyema, mechanical ventilation, and septic shock in a study of 185 patients. In another study performed in patients with severe CAP, a rise in PCT during hospitalization correlated with mortality. In other studies, PCT data have supplemented the information provided by prognostic scoring tools. Krüger et al. [29] found that PCT identified low-risk patients in all severity classes, and that the finding of a low PCT value had a 98.9% negative predictive value for mortality, regardless of the results of prognostic scoring tools. Similarly in a study of 1,651 patients admitted with CAP, there were 546 in high PSI classes (IV and V), but in the 126 of these who had low PCT levels, only 2 died [30], suggesting the advantage of combining serum markers with the commonly used prognostic indices.
Although there are no absolute criteria for severe pneumonia, or need for ICU admission, in the 2007 American Thoracic Society/Infectious Society Diseases of America (ATS/IDSA) guidelines, [4] severe CAP was defined as the presence of one of two major criteria (need for mechanical ventilation, or septic shock requiring pressors) or the presence of three of nine minor criteria. These minor criteria were a PaO2/FiO2 ratio of 250 or less, respiratory rate 30 per minute or more, confusion, multilobar infiltrates, systolic BP less than 90 mm Hg despite aggressive fluid resuscitation, BUN greater than 20 mg per dL, leukopenia (< 4,000 cells per mm3), thrombocytopenia (< 100,000 cells per mm3), and hypothermia (< 36°C) [4]. This approach requires further validation, but in one study of 2,102 patients, of which 235 were admitted to the ICU, this predictive rule had a sensitivity of 71% and a specificity of 88% for determining need for ICU admission. This degree of accuracy was similar to the 2001 ATS guideline rule [31]. The use of only minor criteria to define need for ICU care is uncertain, since in that study, only 47 of 219 patients meeting only minor criteria needed ICU admission, and the presence of minor criteria alone did not increase mortality risk.
CAP Prognostic Factors Defined After Initial Management
The above discussion has focused on data available on admission that can be used to guide the site of care decision. However, after admission, the results of cultures become available, and therapy (accurate or not) is given, and these events can impact prognosis. Garau et al. [32] have found that late and overall CAP mortality are reduced if patients have negative blood cultures, and if antibiotic therapy is given according to guidelines. Among patients with severe CAP, the most important prognostic finding during therapy is radiographic progression [19]. Ineffective initial empiric therapy has also been identified as a potent predictor of death, being associated with a 60% mortality rate, compared with an 11% mortality rate when patients received initial effective therapy [33]. Similarly, in other studies of CAP, the use of a combination of a β-lactam and a macrolide antibiotic was associated with a lower mortality than if other therapies were given [4], and the use of guideline compliant therapy was associated with a reduced duration of mechanical ventilation [34]. In the setting of pneumococcal bacteremia, the use of combination therapy is associated with reduced mortality, compared with monotherapy, particularly for patients treated in the ICU [4]. Not only must initial therapy be accurate, it must be timely, and in patients with septic shock (from all sources, including pneumonia), mortality increases by 7% for each hour in the delay of initiating therapy [35]. Retrospective data have also shown a reduced mortality for admitted CAP patients who are treated within 4 hours of arrival to the hospital compared with those who are treated later [4].
The interaction between prognostic factors related to therapy and bacteriology is most evident when patients with CAP are infected with drug-resistant organisms, particularly drug-resistant pneumococcus. Pneumococcal resistance to penicillin
is increasing and is present in more than 40% of all pneumococci by older definitions of in vitro resistance [4]. Studies have defined the clinical features of patients at risk for drug-resistant pneumococcus (drug-resistant S. pneumoniae [DRSP]) [4] and these include older than 65 years, β-lactam therapy in the past 3 months, multiple medical comorbidities, alcoholism, nosocomial acquisition, and contact with a child in day care. Recently, the criteria for resistance in nonbacteremic infection have changed, and fewer organisms are defined as resistant, compared with the past, since many available therapies are known to be effective [36]. Studies of bacteremic pneumococcal pneumonia have shown that the presence of resistance is not itself a predictor of a poor outcome or a risk factor for mortality. In the absence of meningitis, clinical failure with high-dose β-lactam therapy is currently unlikely [4,36]. Most investigators have found no difference in mortality for patients infected with resistant or sensitive organisms, after controlling for comorbid illness [36,37], although patients with resistant organisms may have a more prolonged hospital stay, and suppurative complications such as empyema [38], and in HIV infection, the presence of high-level penicillin resistance has been associated with increased mortality [39]. The breakpoint for clinically significant penicillin resistance is a minimum inhibitory concentration value of 4 μg per mL or more, with increased mortality in patients with invasive disease (bacteremia) and this degree of resistance, who do not die in the first 4 days of illness [40].
is increasing and is present in more than 40% of all pneumococci by older definitions of in vitro resistance [4]. Studies have defined the clinical features of patients at risk for drug-resistant pneumococcus (drug-resistant S. pneumoniae [DRSP]) [4] and these include older than 65 years, β-lactam therapy in the past 3 months, multiple medical comorbidities, alcoholism, nosocomial acquisition, and contact with a child in day care. Recently, the criteria for resistance in nonbacteremic infection have changed, and fewer organisms are defined as resistant, compared with the past, since many available therapies are known to be effective [36]. Studies of bacteremic pneumococcal pneumonia have shown that the presence of resistance is not itself a predictor of a poor outcome or a risk factor for mortality. In the absence of meningitis, clinical failure with high-dose β-lactam therapy is currently unlikely [4,36]. Most investigators have found no difference in mortality for patients infected with resistant or sensitive organisms, after controlling for comorbid illness [36,37], although patients with resistant organisms may have a more prolonged hospital stay, and suppurative complications such as empyema [38], and in HIV infection, the presence of high-level penicillin resistance has been associated with increased mortality [39]. The breakpoint for clinically significant penicillin resistance is a minimum inhibitory concentration value of 4 μg per mL or more, with increased mortality in patients with invasive disease (bacteremia) and this degree of resistance, who do not die in the first 4 days of illness [40].
Nosocomial Pneumonia in the Intensive Care Unit: Hospital-Acquired Pneumonia
Risk Factors for HAP and VAP
As mentioned, pneumonia is the nosocomial infection most likely to contribute causally to the death of patients, particularly those treated with mechanical ventilation (VAP). Risk factors for this infection fall into four categories: the underlying primary critical illnesses leading to ICU admission; coexisting medical illness; factors associated with therapies that are frequently used in the ICU; and malnutrition. Thus, some of the common conditions associated with nosocomial pneumonia include risk factors present on admission such as immune-suppressive illness, risk of aspiration (coma, impaired consciousness), serious comorbid illnesses (chronic heart or lung disease, renal failure, malignancy, diabetes mellitus), ARDS, malnutrition (serum albumin < 2.2 mg per dL), obesity, older than 60 years, smoking and drug abuse, need for major surgery, and recent major trauma or burns. Other nonmodifiable risk factors that increase the risk of nosocomial pneumonia are treatment related such as prior antibiotic therapy, immune suppressive therapy (including corticosteroids), need for multiple transfusions, transport out of the ICU, mechanical ventilation with PEEP, tracheostomy, nasogastric tube use, supine position in the first 24 hours after admission, and intestinal bleeding prophylaxis [2,41]. The most important risk factor for nosocomial pneumonia is probably mechanical ventilation, explained later in the chapter.
HAP is currently the second most common nosocomial infection in the United States and is associated with high mortality and morbidity [2]. HAP accounts for up to 25% of all ICU infections and for more than 50% of the antibiotics prescribed [2]. The presence of HAP increases hospital stay by an average of 7 to 9 days per patient and VAP leads to an excess cost of more than $40,000 per patient [2]. Available data suggest that it occurs at a rate of between five and ten cases per one thousand hospital admissions, and that critically ill patients who develop VAP appear to be twice as likely to die compared with similar patients without VAP (pooled odds ratio [OR], 2.03; 95% confidence interval, 1.16 to 3.56) [9].
In ICU patients, nearly 90% of episodes of HAP occur during mechanical ventilation, referred to as VAP, when the illness develops after 48 hours of endotracheal intubation and ventilation. Intubation increases the risk of acquiring pneumonia by as much as 6- to 20-fold [2]. Older studies estimated that the risk of nosocomial pneumonia was 1% per day of mechanical ventilation, but other data show a risk of 3% per day for the first 5 days, 2% per day for days 6 to 10 days, and 1% per day for days 11 through 15 [42]. Although VAP occurs in 9% to 27% of all intubated patients [2], since many patients are intubated for only a short time, up to half of all VAP episodes begin within the first 4 days of mechanical ventilation (early-onset pneumonia) [2]. In the past, mortality rates were high in mechanically ventilated patients, with Craven et al. [43] reporting a 55% mortality rate in 49 patients with nosocomial pneumonia treated with mechanical ventilation, and Bryan and Reynolds [44] finding a 58% mortality rate in patients with bacteremic nosocomial pneumonia.
Recently, in the United States, there has been a focus on prevention of VAP, through the use of “ventilator bundles,” and there have been numerous reports of “zero VAP” as a consequence of these efforts [45]. These bundles, which include daily assessment for weaning, daily interruption of sedation, elevation of the head of the bed, prophylaxis of deep vein thrombosis, and gastrointestinal bleeding, have been quite successful, but most studies have reported a reduction in the frequency of VAP, without associated reductions in mortality or antibiotic use. Thus, it is unclear if VAP is really being prevented, or if the disease is being diagnosed less often, especially given the subjective nature of the VAP definition, and the possibility of treating patients for another diagnosis. If VAP does occur, proper management can impact outcome, with several studies showing that management with a guideline-concordant therapy can improve outcomes [46,47].
The relation between pneumonia and ARDS is particularly interesting. In older studies, as many as one third of all cases of ARDS were the result of pneumonia [48], but secondary pneumonia was the most common nosocomial infection acquired by patients with established ARDS [2,11]. When patients with ARDS develop pneumonia, it is generally a late event, occurring after at least 7 days of mechanical ventilation [2], and when it occurs [11], it can be the start of a progressive downhill course characterized by multiple organ failure. In a European collaborative study of 583 patients with ARDS, pneumonia was the cause in 33% of cases and a complication in 34% [49]. When quantitative diagnostic methods were used in ARDS patients, Chastre et al. [50] found an incidence of 55% of VAP in patients with ARDS; however, there were no significant changes in survival between ARDS patients with VAP and those without VAP. All patients who developed VAP had a significantly longer duration of mechanical ventilation, regardless of whether they had coexisting ARDS.
Healthcare-Associated Pneumonia
The other type of pneumonia that has been described recently and may require ICU admission is HCAP. This entity refers to patients who develop infection while having contact with the healthcare environment, such as those residing in nursing homes, those treated in dialysis units, patients who have been in the hospital in the past 90 days, and those getting home infusion therapy of home wound care (Table 68.2), and some of these patients are at risk for infection with multidrug resistant (MDR) organisms. This has also been addressed in the ATS/IDSA guidelines as a form of HAP [2]. However, recent studies have shown that patients who qualify has having HCAP are a heterogeneous population, and that when HCAP patients
are managed in the ICU, the frequency of MDR pathogens is much greater than when they are not as ill and do not need ICU care [51].
are managed in the ICU, the frequency of MDR pathogens is much greater than when they are not as ill and do not need ICU care [51].
Table 68.2 Hospital-Acquired Pneumonia and Healthcare-Associated Pneumonia: Risk Factors for Multidrug Resistant Organisms | ||
---|---|---|
|
Pathogenesis of Pneumonia
Normal Host Defenses
When an organism enters the respiratory tract, it encounters a host defense system designed to repel and remove it from every anatomic site in the airway. Pneumonia develops when the size of the organism inoculum overcomes the host defense system, when the organism is so virulent that it cannot be repelled, or when the patient is so impaired that he or she is unable to resist an organism type or inoculum size that could ordinarily be handled by a fully functioning host defense system.
The oropharynx is ordinarily free of enteric Gram-negative bacilli because salivary proteases, secretions, and local immunoglobulin-A (IgA) antibody prevent these bacteria from establishing a foothold on the mucosal surface. The intrinsic ability of the oral epithelium to bind or adhere to Gram-negative bacteria is poor in healthy people [52]. With a variety of acute illnesses, such as malnutrition, uremia, and general surgery, Gram-negative bacteria can bind more avidly to the oral epithelium, and colonization can occur [52].
The lower respiratory tract (starting beneath the vocal cords) has a complex host defense system that keeps this site sterile in normal people [2,3]. For this area to become infected, organisms must overcome the physical barrier of the vocal cords and the tracheobronchial protective mechanisms of cough, bronchoconstriction, airway angulation, and the upward transport of the mucociliary blanket [3]. As in the oropharynx, bacterial adherence is necessary for Gram-negative bacteria to colonize the tracheobronchial tree. Protective substances in respiratory secretions include IgA, the predominant immunoglobulin of the upper airway; IgG, which dominates in the lower respiratory tract; complement; lysozymes; surfactant; and fibronectin [3]. The resident phagocytic cell of the lower respiratory tract is the alveolar macrophage, but its function can be augmented by the production of inflammatory cytokines which can promote the recruitment of blood neutrophils and the development of cell-mediated and humoral immunity.
In the setting of focal lung infection, the cytokine inflammatory response is normally localized to the site of initial infection [53]. Severe pneumonia occurs when the inflammatory response is unable to be localized (due to overwhelming infection or inappropriate bilateral inflammation) or if the inflammatory response extends to the systemic circulation (sepsis syndrome). The innate immune response of the lung is organized to recognize pathogens and once this occurs, a cytokine response follows. Pathogen recognition is mediated by toll-like receptors for Gram-negative bacteria or by pathogen-stimulated production of interleukin 1 (IL-1) or tumor necrosis factor (TNF). Once pathogens are recognized through these mechanisms, nuclear factor κ B is produced by inflammatory cells, which in turn leads to cytokine production that can recruit more inflammatory cells. In addition, the lower airway handles individual pathogens in specific ways. For example, S. aureus is removed by resident alveolar macrophages, whereas certain enteric Gram-negative bacteria and the pneumococcus require the recruitment of neutrophils (presumably in response to interleukin-8) to be cleared [53]. Cell-mediated immunity is required to resist infection with L. pneumophila and M. tuberculosis. Viruses are handled somewhat differently from bacteria, and important factors in defense against these agents include the alveolar macrophage, neutralizing antibodies (IgG, IgA, IgM), cytotoxic T lymphocytes, and cytokines such as interferon. Specific genetic immune impairments or acquired immune dysfunction can cause specific aspects of the inflammatory response to malfunction and lead to infection with specific, predictable pathogens.
How Microorganisms Reach the Lung
Bacteria and other infectious agents can reach the lung by inhalation from ambient air, hematogenously from distal sites of infection, by direct extension or exogenous penetration, and by aspiration from a colonized oropharynx and nasopharynx [2,3]. Inhalation is an uncommon route of organism entry except for pathogens such as L. pneumophila, viruses, and M. tuberculosis. Hematogenous spread can occur with septic emboli from such sites as the valves of the right cardiac chambers. Exogenous penetration is an unlikely route of bacterial entry but can occur, for example, with extension of an abdominal infection into the pleural space and then the lung parenchyma. Most pneumonias result when microorganisms are aspirated from a previously colonized oropharynx [2]. Nosocomial pneumonia is frequently preceded by Gram-negative bacillary colonization of the oropharynx [2]. The source of bacteria that colonize the upper airway is most likely the patient’s own lower intestinal flora, but the nasal sinuses and stomach can also harbor bacteria that can subsequently reach the lung. The coexistence of nosocomial sinusitis and pneumonia has been documented, often with the same organisms, and both infections can be promoted by the presence of a nasogastric or nasotracheal tube [2].
In addition to promoting sinusitis, the endotracheal tube and the nasogastric tube can also serve as additional pathways for bacterial entry to the lung. Insertion of an endotracheal tube allows organisms direct access to the lung from the hands of the ICU staff, thereby avoiding the defense mechanisms present above the vocal cords. Any organisms that reach the inside of the endotracheal tube can proliferate to large numbers because this site is free from host defenses, and a biofilm commonly lines the interior of the endotracheal tube and can contain as many as 106 organisms per cm of the tube surface [54]. These organisms can reach the lung every time an intubated patient is suctioned [2]. Recently, this problem has been addressed by
developing new materials for endotracheal tubes that are antibacterial, one such device is a silver-coated endotracheal tube, which may be able to reduce the incidence of VAP, but has had no impact on mortality [55]. Another factor in VAP pathogenesis is the ventilator circuit tubing, which can easily be contaminated by large numbers of bacteria [2]. Interestingly, the ventilator circuits are usually not contaminated by the ventilator but rather by the patient, as the circuit becomes colonized in large numbers, as bacteria proliferate in the water condensate in the tubing. If handled carefully, the circuits are not a major source of pneumonia pathogens, and the incidence of pneumonia is not increased even if ventilator circuit tubing is never changed during the course of therapy [2]. The presence of an endotracheal tube can promote infection, and studies [2] have shown that when an endotracheal tube is present, some bacteria, particularly P. aeruginosa, can colonize the lower airway directly without first colonizing the oropharynx.
developing new materials for endotracheal tubes that are antibacterial, one such device is a silver-coated endotracheal tube, which may be able to reduce the incidence of VAP, but has had no impact on mortality [55]. Another factor in VAP pathogenesis is the ventilator circuit tubing, which can easily be contaminated by large numbers of bacteria [2]. Interestingly, the ventilator circuits are usually not contaminated by the ventilator but rather by the patient, as the circuit becomes colonized in large numbers, as bacteria proliferate in the water condensate in the tubing. If handled carefully, the circuits are not a major source of pneumonia pathogens, and the incidence of pneumonia is not increased even if ventilator circuit tubing is never changed during the course of therapy [2]. The presence of an endotracheal tube can promote infection, and studies [2] have shown that when an endotracheal tube is present, some bacteria, particularly P. aeruginosa, can colonize the lower airway directly without first colonizing the oropharynx.
The gastrointestinal tract, particularly the stomach, can serve as a reservoir for bacteria, and several investigators have shown that Gram-negative bacilli can move retrograde from the stomach to the oropharynx and then antegrade into the lung [56]. The stomach can be the source of 20% to 40% of the enteric Gram-negative bacteria that colonize the trachea of intubated patients [56], but it is difficult to determine if these colonizing gastric bacteria also lead to pneumonia. One of the ways that the stomach can be an important source of pneumonic organisms is through the mechanism of reflux and aspiration. When a nasogastric tube is used for feeding, it can promote aspiration, especially if a large-bore tube is used with a bolus feeding method rather than with a continuous infusion of enteral nutrients, and if the patient is kept in a supine position [57]. When a nasogastric tube is present, it may promote pneumonia if the gastric contents have a pH above 4 to 6, as can occur with the use of antacids, H2 blockers, and enteral feeding. An elevated pH can increase the number of Gram-negative bacteria in the stomach, increases to as many as 1 to 100 million per mL of gastric juice, and elevation of gastric pH has been reported as a risk factor for nosocomial pneumonia, although not in all studies [43,58]. The Canadian Critical Care Trials Group reported that acidified enteral feeds preserve gastric acidity and substantially reduce gastric colonization in critically ill patients; however, in this study, there was no impact on the incidence of pneumonia with this intervention [58]. Increases in gastric volume can be detrimental and promote aspiration, thus accounting for the observation that when continuous enteral feeding leads to an elevation of gastric pH (and presumably an elevation of gastric volume), the incidence of pneumonia is higher than when continuous feeding is used but does not raise pH [59]. Placing the feeding tube into the jejunum to avoid an elevation in gastric pH did not reduce the risk of pneumonia [60]. Another way to minimize the impact of the stomach and to avoid aspiration is to keep patients in a semierect position whenever possible, particularly because the supine position can favor aspiration when a nasogastric tube is in place [57].
Airway Colonization and Nosocomial Pneumonia
Colonization (the persistence of organisms in the absence of a host response and without an adverse effect to the host) of the respiratory tract by enteric Gram-negative bacilli is the first step toward the development of nosocomial pneumonia [2]. Risk factors for Gram-negative colonization of the upper and lower respiratory tract are similar and include antibiotic therapy, endotracheal intubation, smoking, malnutrition, general surgery, and therapies that raise gastric pH [3,52]. Additional risk factors for oropharyngeal colonization include azotemia, diabetes, coma, hypotension, advanced age, and underlying lung disease [52]. Additional risk factors for tracheobronchial colonization include chronic bronchitis, cystic fibrosis, ciliary dysfunction, tracheostomy, bronchiectasis, acute lung injury, and viral infection [52]. The distinction between colonization and infection in mechanically ventilated patients is less clear than in the past, with recognition and focus on ventilator-associated tracheobronchitis (VAT) [61]. Some patients who are mechanically ventilated can have high concentrations of pathogenic organisms in the tracheobronchial tree, in the absence of pneumonia, yet some may be clinically ill, and therapy could potentially prevent some from progressing to VAP.
One pathogenetic mechanism that links many of the clinical risk factors for upper and lower airway colonization is a cell–cell interaction termed bacterial mucosal adherence. Many clinical disease states can alter the oropharyngeal or tracheal epithelium, making the cell surface more receptive for binding by such bacteria as P. aeruginosa [52]. Diseases that result in an increased number of oropharyngeal and tracheal cell bacterial receptors are many of the same processes that promote colonization of these sites [52]. One study of intubated patients demonstrated the rapidity with which the endotracheal tube itself became colonized with enteric Gram-negatives and found that colonization took place despite the use of bacterial filters in the ventilator circuit [62]. Colonization is a common finding in intubated patients, and the presence of potential pathogens in the respiratory secretions of intubated patients is to be expected, and does not require therapy unless there are clinical signs of infection.
Host Defense Impairments in Acute and Chronic Illness that Predispose to Pneumonia
Many systemic diseases increase the risk of pneumonia as a result of disease-associated malfunctions in the respiratory host defense system, including ARDS, sepsis, CHF, malnutrition, renal failure, diabetes mellitus, chronic liver disease, alcoholism, cancer, and collagen vascular disease [63]. For example, sepsis can lead to a number of inflammatory events that interfere with respiratory tract immune defenses. In addition, many illnesses can be complicated by pneumonia because they require therapy with medications that interfere with immune function. Several studies have shown that acute and chronic malnutrition (Table 68.3) can increase the risk of bacterial and viral infections both in and out of the hospital. Genetic polymorphisms may explain why patients who have certain inherited patterns of immune response are more prone to severe forms of pneumonia than others, and even mortality. CAP severity is increased with genetic changes in the IL-10-1082 locus that are often present along with changes in the TNF-α-308 locus. Another genetic change associated with an increased risk of septic shock from CAP is a modification in heat shock protein 70-2
[64]. Currently, there are large number of genes that can affect the severity and outcome of CAP, but the clinical application of this is not defined. One recent observation about gender differences in the immune response was that men have a higher degree of systemic inflammation on admission for CAP (higher levels of TNF, IL-6, and IL-10), and that higher levels of these mediators increased pneumonia mortality risk [65].
[64]. Currently, there are large number of genes that can affect the severity and outcome of CAP, but the clinical application of this is not defined. One recent observation about gender differences in the immune response was that men have a higher degree of systemic inflammation on admission for CAP (higher levels of TNF, IL-6, and IL-10), and that higher levels of these mediators increased pneumonia mortality risk [65].
Table 68.3 Lung Host Defense Impairments with Malnutrition | |
---|---|
|
Etiology of Pneumonia
Community-Acquired Pneumonia
Even with extensive diagnostic testing, a specific etiologic agent can be identified in only approximately 50% of pneumonias that develop outside of the hospital, although the rate of pathogen recovery may be higher in intubated and mechanically ventilated patients [66,67]. Although the exact incidence of viral pneumonias is unknown, these agents may account for up to one third of all community-acquired cases. The most common pathogen identified in pneumonias arising out of the hospital is the pneumococcus, followed by M. pneumoniae, L. pneumophila, H. influenzae, C. pneumoniae, anaerobes, S. aureus, and enteric Gram-negative bacilli, although the exact incidence of each pathogen varies depending on a number of factors. These include the severity of the acute illness, the age of the patient, and the types of comorbidity present in a given patient population [4,67]. In the elderly, although pneumococci are still the most common pathogens, enteric Gram-negative organisms may be responsible for 20% to 40% of all cases of pneumonia, and anaerobes and H. influenzae are other common agents [68]. However, age alone has little impact on the bacterial etiology of CAP, but rather, the comorbid illnesses that become more common in the elderly affect bacteriology [69]. The most common CAP pathogens leading to ICU admission (severe pneumonia) are pneumococcus, L. pneumophila, epidemic viruses (influenza), S. aureus (including MRSA), and enteric Gram-negative bacilli, including, in some patients, P. aeruginosa [4,67,68]. The incidence of CAP caused by community-acquired MRSA (CA-MRSA) is on the rise, but the exact frequency and the impact on mortality and other outcomes remain to be defined [70]. Although Gram-negatives are more common in VAP and HCAP than in CAP, risk factors for Gram-negative pneumonia (in addition to nursing home residence, an HCAP risk factor) are cardiac disease, smoking history, and clinical features of severe illness including hyponatremia, septic shock, and severe tachypnea [71].
Table 68.4 Likely Pathogens for Pneumonia in the Critically Ill | ||||||||||||||||||||||||||||||
---|---|---|---|---|---|---|---|---|---|---|---|---|---|---|---|---|---|---|---|---|---|---|---|---|---|---|---|---|---|---|
|
Other pathogens that can lead to respiratory failure include H. influenzae, pathogens associated with aspiration (such as anaerobes), P. jiroveci, tuberculosis, varicella, and respiratory syncytial virus. Mixed infection occurs in more than 10% of patients with CAP requiring hospitalization, and in one study, in patients with mixed CAP, S. pneumoniae was the most prevalent microorganism (44 out of 82; 54%) [72]. In that study, the most frequent combination was S. pneumoniae with H. influenzae (17 out of 82; 21%), and influenza A occurred with S. pneumoniae in 5 out of 28 (18%). Of note, patients with mixed pyogenic pneumonia more frequently developed shock when compared with patients with single pyogenic pneumonia (18% vs. 4%) [72].
When evaluating a patient with pneumonia, it is important to understand the status of each individual’s respiratory host defense system to predict which possible pathogen is most likely (Table 68.4). Thus, CAP in a previously healthy person is most likely due to a pathogen of such intrinsic virulence that it can overcome even an intact host defense system. These pathogens include S. pneumoniae, Legionella sp, S. aureus, and M. pneumoniae. Certain agents should be suspected in specific clinical settings. If the patient has a serious underlying illness, then organisms of less intrinsic virulence that would ordinarily be eliminated by a normal host can be responsible. When an alcoholic has pneumonia, anaerobes and Klebsiella pneumoniae become more likely; those with chronic bronchitis may be infected with nontypeable H. influenzae and Moraxella catarrhalis; cardiac patients commonly have pneumococcal infection; those with cystic fibrosis are commonly infected by S. aureus and P. aeruginosa; and those with risk factors for aspiration can have enteric
Gram-negative bacterial or anaerobic lung infection. Other associations are listed in Table 68.4. Certain historical information can be valuable, such as an appropriate travel or exposure history that suggests specific etiologic pathogens (Table 68.5).
Gram-negative bacterial or anaerobic lung infection. Other associations are listed in Table 68.4. Certain historical information can be valuable, such as an appropriate travel or exposure history that suggests specific etiologic pathogens (Table 68.5).
Table 68.5 Historical and Physical Features Useful in Pneumonia Diagnosis | ||||||||||||||||||||||||||||
---|---|---|---|---|---|---|---|---|---|---|---|---|---|---|---|---|---|---|---|---|---|---|---|---|---|---|---|---|
|
Nosocomial Pneumonia
Both VAP and HCAP may be caused by a variety of Gram-positive and Gram-negative bacteria, many of which are multidrug resistant (MDR). These infections may be polymicrobial and are seen more often in patients with ARDS than in other ventilated patients [73]. Viral or fungal pathogens are rarely causative in immunocompetent hosts [74,75]. Nosocomial viral infections can occur if infected staff members come to work when their illness is incubating. Common pathogens include aerobic Gram-negative bacilli, such as P. aeruginosa, Escherichia coli, K. pneumoniae, and Acinetobacter species. Gram-positive infections include S. aureus, particularly MRSA, which has been increasing in the United States [76]. Pneumonia due to S. aureus is more common in patients with diabetes mellitus, head trauma, and ICU patients. In the National Nosocomial Infections Surveillance (NNIS) system data examining changes in the organisms from 1986 to 2003, Gram-negative aerobes persisted as being the most frequent organisms in HAP (65.9%), with little change in their distribution over this period, except for a rise in the proportion of Acinetobacter in 2003, from 1.5% in 1975 to 6.9% in 2003. The commonest Gram-negative organism reported was Pseudomonas (18.1%), and others included Klebsiella spp (7.2%), Acinetobacter spp (6.9%), and E. coli (5%). The Gram-positive organisms included S. aureus (included Enterobacter spp (10%), 27.8%), coagulase-negative Staphylococcus (1.8%), and Enterococci (1.3%) [77]. Among patients who have a prolonged hospital stay, therapy with corticosteroids or antibiotics, need for long-term mechanical ventilation, and in those with ARDS, the pathogen most likely to cause pneumonia is P. aeruginosa, but in many hospitals, Acinetobacter is becoming an increasing concern. Contamination with Legionella sp in the water system can lead to infection, especially if patients are being treated with corticosteroids. Another pathogen that should be considered when nosocomial pneumonia arises in the setting of corticosteroid therapy for COPD is Aspergillus sp. [78]. HAP involving anaerobic organisms may follow aspiration in nonintubated patients but is rare in patients with VAP [2]. Gram-negative organisms are more common with aspiration, especially in the healthcare environment, including the nursing home [51,68]. Oropharyngeal commensals such as viridans group streptococci, coagulase-negative staphylococci, Neisseria species, and Corynebacterium species can produce infection in immunocompromised hosts and some immunocompetent patients [2]. The identity of specific MDR pathogens causing HAP varies from one ICU to another, and depends on the patient population treated and the degree of prior antibiotic exposure, but the dominant organisms change over time [2,77]. Risk factors for infection with MDR pathogens are summarized in Table 68.2.
Elderly patients represent a diverse population of patients with pneumonia, particularly HCAP. Elderly residents of long-term care facilities have been found to have a spectrum of pathogens similar to late-onset HAP and VAP [2,51,79].
In patients aged 75 years and older with severe pneumonia, El-Solh et al. found S. aureus (29%), enteric Gram-negative rods (15%), S. pneumoniae (9%), and Pseudomonas species (4%) as the most frequent causes of nursing home–acquired pneumonia [74]. In this population, MDR organisms are most likely in patients with a history or prior antibiotic therapy and poor functional status. Scant data are available about HAP in patients who are not mechanically ventilated. In general, the bacteriology of nonventilated patients is similar to that of ventilated patients, including infection with MDR pathogens. The frequency of resistant Gram-negative bacilli is often high enough in nonventilated patients that they should be accounted for in designing an empiric therapy regimen. Sopena et al. [80] looked at a multicenter population of non–ICU admitted patients with HAP in Spain and described that the most common etiologies were S. pneumoniae, L. pneumophila, Aspergillus sp, P. aeruginosa, and several Enterobacteriaceae. Another study therapy for nonsevere HAP and HCAP [81] found among 303 patients, 53.5% had an identifiable etiology, with Enterobacteriaceae in 19.5%, followed by S. pneumoniae in 12.9% and S. aureus in 11.6%. In a study that examined both HAP and VAP in the same hospital, patients with VAP had infection with non-Enterobacteriaceae Gram-negatives (P. aeruginosa and Acinetobacter spp) more commonly than HAP patients, while S. pneumoniae was more common in HAP patients [82].
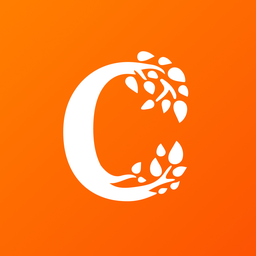
Full access? Get Clinical Tree
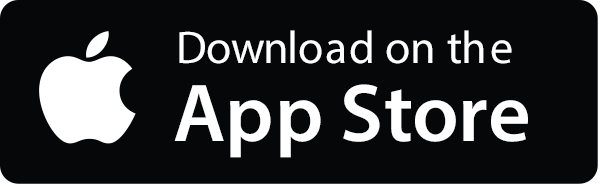
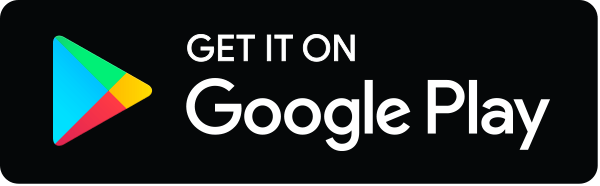