The central focus of treating acid–base disturbances is the understanding of the biochemistry of the hydrogen ion. Hydrogen ion concentrations in the various body fluid compartments are precisely regulated in the face of enormous variations in local production and clearance. Deviations in hydrogen ion concentrations from the normal range can cause marked alterations in protein structure and function, enzyme activity, and cellular function. Although hydrogen ions are continuously produced in the hydrolysis of adenosine triphosphate, the largest contribution of metabolic acids arises from the oxidation of carbohydrates, principally glucose, to produce carbon dioxide (volatile acid, approximately 24,000 mEq per day). By comparison, the average net production of nonvolatile metabolic acid, such as lactate, is relatively small (approximately 60 mEq per day).
The hydrogen ion concentration is regulated to maintain the arterial blood pH between 7.35 and 7.45. However, expression of the hydrogen ion concentration as pH masks large variations in hydrogen ion concentration despite small changes in pH. For example, a pH range of 7.0 to 7.7 is associated with a fivefold change (100 nmol/L to 20 nmol/L) in hydrogen ion concentration. The pH of venous blood and interstitial fluid is lower than that of arterial blood (approximately 7.35).
Mechanisms for Regulation of Hydrogen Ion Concentration
Regulation of pH over a narrow range depends on (a) buffer systems, (b) ventilatory responses, and (c) renal responses. The buffer system mechanism is local and immediate, but incomplete. Ventilatory responses are slower (minutes) and usually incomplete. Renal responses develop very slowly (hours) but can produce nearly complete pH correction.
Buffer Systems
Body fluids contain acid–base buffer systems that immediately combine with acid or alkali to prevent excessive changes in the hydrogen ion concentration. This ability to neutralize excess protons maintains the local pH near 7.4 in the face of continuous acid generation. The most important buffer systems are (a) bicarbonate and carbonic acid in plasma, interstitial and intracellular fluid, and bone; (b) hemoglobin and other proteins in intracellular fluid; (c) plasma proteins; and (d) phosphates in intracellular and extracellular fluid and the kidney (Fig. 26-1).

Bicarbonate Buffering System
The bicarbonate buffering system consists of carbonic acid (H2CO3) and sodium bicarbonate (NaHCO3). Bicarbonate buffer is primarily a product of the approximately 200 mL of carbon dioxide produced per minute, of which considerably less than 1% dissolves to become carbonic acid. Carbonic acid is a weak acid because of its limited degree of dissociation (<5% at physiologic pH) into hydrogen and bicarbonate ions (Fig. 26-2). Most carbonic acid in solution almost immediately dissociates into carbon dioxide and water, the net result being a very high concentration of dissolved carbon dioxide compared to the concentration of bicarbonate ions. This relationship is described mathematically by the Henderson-Hasselbalch equation, which can be used to calculate the pH of a solution if the concentration of bicarbonate ions and dissolved carbon dioxide is known (Fig. 26-3).


The addition of a strong acid such as hydrochloric acid to the bicarbonate buffering system results in conversion of the strong acid to weak carbonic acid (Fig. 26-4). Therefore, a strong acid lowers the pH of body fluids only slightly. The addition of a strong base, such as potassium hydroxide, to the bicarbonate buffering system results in the formation of a weak base and water. Buffers are most effective when they operate at a pH that is close to their pKa (under these circumstances, the buffer system is approximately 50% dissociated). The bicarbonate buffering system is not a powerful buffer because its pKa of 6.1 differs greatly from the normal pH of 7.4. Physiologically, buffers are most effective when their pKa is equal to normal pH. However, the bicarbonate system is important because (a) bicarbonate is present in significant quantities in nearly all fluid compartments, (b) the concentration of its components is ultimately regulated by the lungs and kidneys, and (c) in severe acidosis the pH approaches the pKa of the bicarbonate system thus increasing its efficiency.

The bicarbonate buffer system accounts for >50% of the total buffering capacity of blood. Approximately one-third of the bicarbonate buffering capacity of blood occurs within erythrocytes. The electrical charge of bicarbonate ions limits their diffusion into cells other than erythrocytes.
Hemoglobin Buffering System
Hemoglobin is a particularly effective buffer because it is localized in quantity in erythrocytes; it has a pKa of 6.8 and has a buffering capacity that varies with oxygenation. The imidazole ring of the amino acid histidine has a pKa that is close to the physiologic pH. Thus, hemoglobin and other histidine-containing proteins are excellent physiologic buffers. Furthermore, deoxygenated hemoglobin is a weaker acid (better proton acceptor) than oxyhemoglobin. Thus, in the systemic capillaries, dissociation of oxyhemoglobin to deoxyhemoglobin facilitates the binding of hydrogen ions produced by the dissociation of carbonic acid. This situation is reversed in the pulmonary circulation where the conversion of deoxyhemoglobin to oxyhemoglobin facilitates the release of hydrogen ions.
Protein Buffering System
Like hemoglobin, other histidine-containing proteins are important intracellular buffers. Proteins are localized in high concentrations within the cell where it is estimated that approximately 75% of all the buffering of body fluids occurs, mostly by proteins. Of particular importance is the local buffering of hydrogen ions by proteins in the mitochondria. Although the relatively low concentration of plasma proteins limits their role as extracellular buffers, hypoproteinemia will further reduce buffering capacity, especially in the critically ill patient.
Phosphate Buffering System
The phosphate buffering system is important in most fluid compartments but is especially important in renal tubules, where phosphate is concentrated. Renal tubular fluid is more acidic than extracellular fluid, bringing the pH of renal tubular fluid closer to the pKa (6.8) of the phosphate buffering system. Phosphate is a very important intracellular buffer because it is the most abundant intracellular anion. Furthermore, the relatively acidic pH of intracellular fluid is closer to the pKa of the phosphate buffering system than is the pH of extracellular fluid.
Intracellular pH Regulation
Although blood pH is commonly measured clinically, it is the intracellular pH (pHi) that is of functional importance. The routine measurement and manipulation of pHi is not possible in current practice. Indeed, during hypothermic cardiopulmonary bypass and hibernation, the pHi in heart and brain tissue appears to be highly regulated despite significant deviations in systemic pH. Cellular metabolism, transmembrane transport, membrane potential generation, cell growth and division, cytoskeletal structure, and contractile function are processes that are crucially dependent on pHi (Table 26-2). Furthermore, the optimal function of several organelles, including lysosomes and mitochondria, require that their local pH is significantly different from the general pHi. Thus there are highly regulated mechanisms to maintain local pHi including intracellular buffer systems and membrane-bound proton transporters. Indeed, the pHi (7.0) is higher than is predicted by the −90mV transmembrane potential (pH 6.8). As in the extracellular compartment, intracellular protons are rapidly bound to weak acids and bases resulting in a low free proton concentration.

Ventilatory Responses
Ventilation is quantitatively the most important mechanism of acid removal, given the enormous daily production of volatile acid compared to nonvolatile acid. Ventilatory responses cannot return pH to 7.4 when a metabolic abnormality is responsible for the acid–base disturbance. This reflects the fact that the intensity of the stimulus responsible for increases or decreases in alveolar ventilation will begin to diminish as pH returns toward 7.4. As a “buffer,” ventilatory responses are able to buffer up to twice the amount of acids or bases as all the chemical buffers combined. However, compensation for extreme metabolic acidosis imposes a significant respiratory burden. If the bicarbonate (HCO3−) is reduced to 10 mmol/L, the carbon dioxide tension must be reduced to 15 mm Hg in order to normalize the pH. Most patients cannot hyperventilate to below 20 mm Hg. Further, it is likely that the insult causing severe metabolic acidosis will also adversely affect respiratory muscle function, thus compromising the respiratory response.
Renal Responses
The day-to-day renal contribution to acid–base regulation is directed toward the conservation of bicarbonate and the excretion of hydrogen ions. Plasma bicarbonate is freely filtered at the glomerulus. Almost all filtered bicarbonate must be reabsorbed from the glomerular filtrate to maintain the normal plasma bicarbonate concentration (25 mEq/L) and plasma pH. Most bicarbonate reabsorption occurs in the proximal convoluted tubule and is facilitated by the presence of carbonic anhydrase in the luminal fluid and is driven by the sodium-potassium-ATPase pump in the peritubular cell membrane. Active sodium ion extrusion from the renal tubular cell into the peritubular circulation favors sodium diffusion from the tubular lumen into the tubular cell in exchange for hydrogen ions. Hydrogen in the renal tubular fluid then combines with filtered bicarbonate to form carbonic acid. Carbonic anhydrase facilitates the dissociation of carbonic acid into water and carbon dioxide that both enter the renal tubular cell. Carbon dioxide and water generate bicarbonate, which enters the peritubular circulation accompanied by sodium. The remaining hydrogen ions are secreted into the lumen in exchange for sodium (Fig. 26-5). Inhibition of carbonic anhydrase by acetazolamide interferes with the reabsorption of bicarbonate ions from renal tubular fluid. As a result, excess bicarbonate ions are lost in the urine and the plasma bicarbonate concentration is decreased.

Hydrogen ions are secreted into renal tubules by epithelial cells lining proximal renal tubules, distal renal tubules, and collecting ducts. At the same time, sodium ions are reabsorbed in exchange for the secreted hydrogen ions and combine with bicarbonate ions in the peritubular capillaries. This process is facilitated by aldosterone. As a result, the amount of sodium bicarbonate in the plasma is increased during the secretion of hydrogen ions into renal tubules. Active hydrogen ion transport is inhibited when the urinary pH drops below 4.0. Thus, hydrogen ions must combine with ammonia and phosphate buffers in the renal tubular lumen to prevent the pH from decreasing below this critical level. Ammonia is generated in the mitochondria of the proximal tubule. Ammonia (NH3) combines with hydrogen ions to form ammonium (NH4+), which is excreted in the urine in combination with chloride ions as the weak acid ammonium chloride (Fig. 26-6). In renal insufficiency, the capacity to generate urinary ammonia is impaired, thus reducing hydrogen ion excretion.

Renal responses that regulate hydrogen ion concentrations do so by acidification or alkalinization of the urine. In the presence of acidosis, the rate of hydrogen ion secretion exceeds the net loss of bicarbonate ion into the renal tubules. As a result, an excess of hydrogen ions is excreted into the urine. In the presence of alkalosis, the effect of the titration process in the renal tubules is to increase the number of bicarbonate ions filtered into the renal tubules relative to the secretion of hydrogen ions. Excess bicarbonate ions are excreted into the urine accompanied by cations, most often sodium.
Extracellular fluid is electroneutral such that the sum of the positive charges of all cations must equal the sum of negative charges of all anions. In the process of altering the plasma concentration of bicarbonate ions, it is mandatory to remove some other anion each time the concentration of bicarbonate ions is increased or to increase some other anion when the bicarbonate concentration is decreased. Typically, the anion that follows changes in the concentration of bicarbonate ions is chloride. As the most abundant extracellular anion, physiologic manipulation of chloride appears to be an important element of pH control. Conceptually, when bicarbonate ions are replaced by chloride ions, the pH will generally tend to decrease as a weak acid (carbonic acid) is replaced by a strong acid (hydrochloric acid).
The value of renal regulation of hydrogen ion concentration is not its rapidity but instead its ability to nearly completely neutralize any excess acid or alkali that enters the body fluids. Ordinarily, the kidneys can remove up to 500 mmol of acid or alkali each day. If greater quantities than this are generated, the kidneys are unable to maintain normal acid–base balance, and acidosis or alkalosis occurs. Even when the plasma pH is 7.4, a small amount of acid is still lost each minute. This reflects the daily production of 50 to 80 mmol of more acid than alkali. Indeed, the normal urine pH of approximately 6.4 is due to the presence of this excess acid in the urine.
Classification of Acid–Base Disturbances
Acid–base disturbances are categorized as respiratory or metabolic acidosis (pH <7.35) or alkalosis (pH >7.45) (Table 26-3).1 An acid–base disturbance that results primarily from changes in alveolar ventilation is described as respiratory acidosis or alkalosis. An acid–base disturbance unrelated to changes in alveolar ventilation is designated as metabolic acidosis or alkalosis. Compensation describes the secondary renal or ventilatory responses that occur as a result of the primary acid–base disturbance.

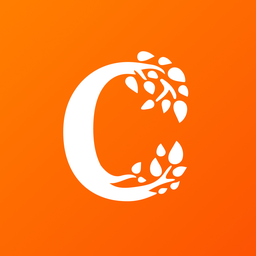
Full access? Get Clinical Tree
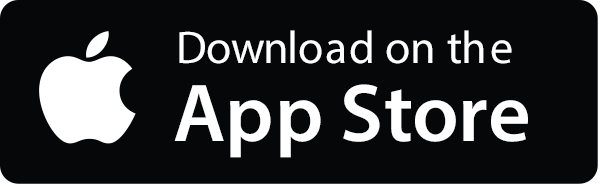
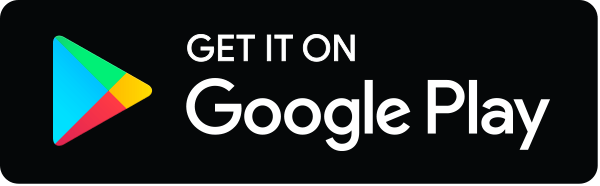