The human body needs to regulate free hydrogen ions (H+) within a narrow window in order to maintain proper protein structure and function. In the process of metabolizing carbohydrates, proteins, and fats, 15,000 mmol of volatile acid, carbon dioxide (CO2), is generated and another 50 to 100 mEq of nonvolatile acid is also produced.1 Despite this, free hydrogen ion concentration is maintained at level of 40 nmol/L (10−6 mmol/L).2 When describing hydrogen concentration of physiologic solutions, we refer to the pH of the solution rather than the actual concentration. The pH of a solution is defined by the following equation:
The pH compatible with human life is in the range of 6.8 to 7.8.3 In order to maintain serum pH in this range, the human body requires the following:2
an effective buffer system to prevent wide fluctuations of pH in response to small additions or subtractions of acid,
the ability to excrete volatile acids (CO2) via the lungs, and
the ability to excrete nonvolatile acids (sulfuric acid, phosphoric acid, and ammonium) via the kidneys.
The bicarbonate/carbon dioxide buffer system is how clinicians usually analyze acid–base balance at the bedside. Carbon dioxide and bicarbonate are easily measured; bicarbonate is important simply because of its high concentration in the blood.
Carbon dioxide effectively becomes an acid when dissolved in solution as described in the following reaction:
The relationship of these reactants can be described by the Henderson-Hasselbalch equation:4
where Paco2 is the partial pressure of CO2 in arterial blood. The amount of CO2 dissolved in solution is proportional to the partial pressure of CO2(Pco2), which is in equilibrium with Paco2 of alveolar air. The solubility constant of CO2 is 0.03.2
The effectiveness of the buffering system can be appreciated when studying this equation. One measure used to quantify strength of acid in solution is the dissociation constant, or pKa. In general, a weak acid will be the most effective buffer when pH is within 1 log of its pKa. Bicarbonate is not an excellent buffer at physiological pH (its pKa is only 6.1). However, because CO2 and bicarbonate can be independently regulated (by the lungs and kidneys, respectively), bicarbonate can be an effective buffer.2 Of course, other extracellular buffers such as phosphates and plasma proteins do contribute to preserving pH; however, they are not considered to be as important. Intracellular buffers, such as hemoglobin, are very important to the initial buffering of respiratory acidosis and alkalosis, and will be discussed later in the chapter.
Although the values change depending on the laboratory or reference, generally, acidemia refers to serum pH less than 7.35 and alkalemia refers to serum pH more than 7.45. We refer to acidosis as the physiologic process that leads to an acidemia, which can be either a fall in bicarbonate or a rise in Paco2. Conversely, alkalosis is due to processes that increase bicarbonate or decrease Paco2. Additionally, an acid–base disturbance is defined as metabolic if the primary process driving it is due to a change in serum bicarbonate, and as respiratory if it’s driven by a change in Paco2. Table 1-1 summarizes these processes.
Acid–Base Disturbance | Appropriate Response |
---|---|
Metabolic acidosis | pH < 7.35 and [HCO3−] < 22 mEq/L |
Respiratory compensation | Paco2 = 1.5 × [HCO3−] + 8 ± 2 mmHg |
Metabolic alkalosis | pH > 7.45 and [HCO3−] > 26 mEq/L |
Respiratory compensation | Paco2 = [HCO3−] + 15 mmHg |
Respiratory acidosis | pH < 7.35 and Paco2 > 45 mmHg |
Acute metabolic compensation (< 24 h) | [HCO3−] increases by 1 mEq/L for every 10-mmHg rise in Paco2 or change in pH = 0.008 × (40 − Paco2) |
Chronic metabolic compensation (> 48 h) | [HCO3−] increases by 4 mEq/L for every 10-mmHg rise in Paco2 or change in pH = 0.003 × (40 − Paco2) |
Respiratory alkalosis | pH > 7.45 and Pco2 < 35 mmHg |
Acute metabolic compensation (< 24 h) | [HCO3−] decreases by 2 mEq/L for every 10-mmHg fall in Paco2 or change in pH = 0.008 × (40 − Paco2) |
Chronic metabolic compensation (> 48 h) | [HCO3−] decreases by 4 mEq/L for every 10-mmHg fall in Paco2 or change in pH = 0.003 × (40 − Paco2) |
Although the lungs can assist in excreting carbonic acid, the 50 to 100 mEq of nonvolatile acid (eg, sulfuric acid and phosphoric acid) that is generated by the body each day must be excreted in the urine. There are 2 steps to excretion of nonvolatile acid:
Reabsorption of the entire filtered bicarbonate load (mostly by the proximal tubule)
Secretion of hydrogen by the distal tubule
Although the nephron can pump protons against an electrochemical gradient, the minimum urine pH that it can generate is about 4.5 to 5. This pH only corresponds to a proton load of about 0.04 mEq/L, which is far less than the 50 to 100 mEq of hydrogen that needs to be excreted each day.2 Hence, most of the free hydrogen that is secreted into the lumen is bound to titratable acids (primarily hydrogen phosphate [HPO42−] or ammonia [NH3]). On a typical diet, 10 to 40 mEq of H+ is excreted bound to titratable acid, and another 30 to 60 mEq is bound to NH3. If more acid needs to be excreted (as in the case of underlying acidosis), then the kidney can generate more NH3 by metabolizing glutamine in the proximal tubule in order to buffer that excess H+ in the urine.
As mentioned above, a pH below 7.35 is considered acidemia. Metabolic acidosis is defined as a pathological process that results in decreased bicarbonate ion concentration. If serum bicarbonate is less than 22 mEq/L, and the serum pH is less than 7.35, then a metabolic acidosis is present.4 In general, there are 3 physiologic mechanisms for metabolic acidosis:
increased generation of acid (eg, diabetic ketoacidosis),
increased bicarbonate loss (eg, diarrhea), and
inability to excrete acid (eg, renal tubular acidosis).1
When there is an increase in serum hydrogen ion concentration due to generation of acid or inability to excrete acid, the following reaction is driven to the right:2
This ultimately leads to consumption of the major extracellular buffer, bicarbonate. If the etiology of acidosis is due to increased bicarbonate loss (as in diarrhea) the reaction is driven to the left, and H+ is ultimately generated in the reaction.
The normal physiologic response to an acid load is to minimize the change in pH via several mechanisms:
extracellular buffering (by serum bicarbonate),
intracellular and bone buffering (by hemoglobin and phosphate from bone), and
respiratory compensation (increase in ventilation to excrete Paco2).
These intracellular and extracellular buffer mechanisms are particularly important to prevent excessive drops in serum pH. Up to 60% of acid loads will be taken up by intracellular and bone buffers. For example, if 12 mEq of hydrogen ions is added to serum, the serum bicarbonate will only fall by a total of 5 mEq because of intracellular and bone buffering.5
The fall in serum pH leads to stimulation of peripheral and central chemoreceptors, which are located on the ventrolateral medullary surface and carotid and aortic bodies, respectively. This stimulation results in a rise of alveolar ventilation by increasing tidal volume and respiratory rate. The increased respiratory response typically begins within 1 to 2 hours and results in a fall in Paco2. The fall in Paco2 in response to metabolic acidosis will approximately follow the Winter equation:
If the Paco2 is higher than what is predicted by the above equation, then it suggests an additional respiratory acidosis. Conversely, a Paco2 that is lower than predicted suggests a simultaneous respiratory alkalosis.
Once a metabolic acidosis has been identified and the respiratory compensation is calculated, the next step in acid–base analysis involves calculating the anion gap (AG). The serum anion gap is defined as
Of course, there is no true electrolyte gap, as the law of electroneutrality dictates that all cations must be equal to all anions. It is inconvenient to measure all the anions in every patient, particularly given how small in concentration some electrolytes are. Since sodium, chloride, and bicarbonate are present in very large concentrations in the serum and can display the largest variability, we can monitor them and identify the presence of unmeasured anions (UA−) if the anion gap rises. The typical unmeasured ions include lactate, phosphate, citrate, sulfate, and, most importantly, albumin. Accumulation of 1 of these UA− causes an increase in the anion gap because of the buffering by bicarbonate of hydrogen produced by these anions:2
Hence, bicarbonate is consumed when buffering the excess hydrogen, leaving only the sodium and UA−. Chloride concentration remains unchanged in this equation, and therefore, the calculated anion gap will rise.
Albumin accounts for about 75% of the anion gap, so falls in albumin must be accounted for when the calculation is made.4 The “normal” anion gap in most labs runs in the range of 3 to 12 mEq/L; the typical correction for low albumin is to add 2.5 mEq/L to the calculated anion gap for every drop of albumin of 1 g/dL (Fig. 1-1).
It should be noted that an abnormally low anion gap can occur in scenarios where there is an excess of unmeasured cation (UC+), which is commonly seen in multiple myeloma (the monoclonal protein is positively charged), lithium toxicity, or hypercalcemia. A negative anion gap occurs in bromide or iodide toxicity because they cause a pseudohyperchloremia.4
When an anion gap acidosis is identified, the next step is to ensure that there is not a mixed metabolic acid–base disturbance. Because there is a predictable relationship between the fall in bicarbonate and the increase in the anion gap, the clinician can identify a concurrent non-gap acidosis or metabolic alkalosis by analyzing the ratio of the increase in the anion gap compared to the fall in serum bicarbonate. This is referred to as the delta-delta.4 For example, in diabetic ketoacidosis, the increase in the anion gap compared to the fall in bicarbonate should be close to 1 to 1. In diabetic ketoacidosis, if the change in the anion gap is significantly greater (5 mEq is the typical error range) than the fall in bicarbonate, we expect a concomitant metabolic alkalosis to be present. Similarly, if the change in bicarbonate is more than 5 mEq greater than the change in the anion gap, then we would predict a mixed non-gap acidosis to be present as well. So, the equation for diabetic ketoacidosis is the following:
In lactic acidosis, the ratio is 0.6 (ie, for every 1 mEq/L rise in the anion gap, the serum bicarbonate falls by 0.6 mEq/L). This difference is probably due to the lower renal clearance of lactate as compared to ketoacids. Hence, the formula for lactic acidosis would be adjusted slightly:5
There is a wide differential diagnosis that has to be considered when an elevated anion gap is diagnosed. The most common categories are lactic acidosis, ketoacidosis (eg, diabetic, alcoholic, starvation induced), uremic acidosis, and drug or toxin ingestion. A helpful mnemonic to remember the different etiologies is GOLD MARRK (Table 1-2).4
Lactic acid is produced from the metabolism of pyruvic acid during the process of anaerobic glycolysis. The digestive tract is responsible for over 50% of the body’s lactic acid production.4,5 Skeletal muscle, brain tissue, skin, and red blood cells (RBCs) also produce lactate, which is metabolized by both the liver and the kidneys. Causes of lactic acidosis can be categorized by subtype (A or B), depending on the mechanism.6 Type A lactic acidosis is the result of decreased tissue perfusion or decreased oxygen delivery that occurs in shock or carbon monoxide toxicity. Anaerobic glycolysis is increased in these conditions, resulting in higher levels of lactic acid. In the setting of shock, reduced perfusion of the liver results in a simultaneous decrease in lactate metabolism. Type B lactic acidosis occurs when mitochondrial or liver function is impaired. The conversion of lactate to pyruvate requires adequate liver and mitochondrial function. If either of these is impaired, lactic acid may accumulate. Metformin and certain antiviral medications (such as zidovudine or stavudine) also can inhibit mitochondrial function. Cyanide toxicity results in type B lactic acidosis because cyanide binds the final enzyme of the mitochondrial cytochrome complex (ie, the electron transfer chain), interrupting normal mitochondrial oxidative phosphorylation.6
Ketoacidosis occurs when glucose is not available to cells due to a lack of insulin, glucose depletion, or cellular dysfunction. The 2 major types of ketoacidosis that are seen in clinical practice are diabetic ketoacidosis and alcoholic/starvation ketosis. In these conditions, there are 2 mechanisms that result in the development of ketoacidosis:
increase in free fatty acid delivery due to increased lipolysis, and
change in hepatocyte function so that free fatty acids are converted to ketoacids and not triglycerides.2,5
During insulin deficiency (which occurs in type 1 diabetes or in starvation states), fatty acids undergo lipolysis. High serum glucagon causes fatty acyl coenzyme A (CoA) molecules within hepatocytes to be converted to ketones (acetoacetate and β-hydroxybutyrate).2,5 These ketones are preferentially taken up and oxidized by the brain and kidneys. In patients with starvation or alcoholic ketosis, the rate of uptake of ketones by these organs approximates the rate of generation, and hence, acidosis tends not to be as severe.7 The presence of low levels of insulin in starvation limits ketosis to some degree. A more detailed discussion of diabetic ketoacidosis can be seen in Chapter 22.
It should be noted that the major type of ketone synthesized is dependent on etiology of the patient’s ketoacidosis. The concentrations of each ketone are based on cellular reduction-oxidation (redox) levels, or in other words, the ratio of nicotinamide adenine dinucleotide phosphate (NADP) to NADPH (the reduced form) in the body. In diabetic ketoacidosis, the average β-hydroxybutyrate–acetoacetate ratio is 5 to 2. In alcoholic ketoacidosis, the ratio is 20 to 1.5 Notably, urine dipstick testing measures acetoacetate and β-hydroxybutyrate, whereas blood serum ketone levels register only β-hydroxybutyrate (Fig. 1-2).
There are several toxins and drugs that increase levels of endogenous acids upon ingestion. In the case of aspirin (acetylsalicylic acid), the therapeutic range in the serum is usually 20 to 35 mg/dL. When levels exceed 40 to 50 mg/dL, patients will present with signs of intoxication.5 Early clinical symptoms include tinnitus, vertigo, nausea, vomiting, and diarrhea. Severe overdose can cause hyperthermia, altered mental status, coma, and death.8 The major anions that accumulate in salicylate poisoning are ketoacids and lactic acid, as salicylate concentrations in serum are very small and do not significantly contribute to the anion gap.5 Salicylate toxicity stimulates respiratory centers in the brainstem, leading to a respiratory alkalosis in addition to the anion gap acidosis.
The mainstay of management of salicylate toxicity includes administration of intravenous sodium bicarbonate to alkalinize the serum to a pH of 7.5 to 7.55. Salicylic acid diffuses easily into central nervous system (CNS) tissue, whereas salicylate ions can be trapped in alkaline serum and urine, and excreted.8,9 In a patient who presents with severe neurologic symptoms, renal failure, or fluid overload, hemodialysis should be initiated. Because salicylate is a small molecule with a small volume of distribution, hemodialysis is very effective to enhance elimination of the drug and should be continued until serum levels are below 20 mg/dL.9
Acetaminophen is another common over-the-counter medication that can cause an elevated gap acidosis in patients who are chronic users and simultaneously malnourished. The metabolic acidosis is secondary to the build-up of pyroglutamic acid.4
Methanol and ethylene glycol are toxic alcohols available in automotive antifreeze and commercial solvents that, when ingested, are metabolized by alcohol dehydrogenase and aldehyde dehydrogenase (the enzymes that metabolize alcohol) into toxic metabolites. Methanol, which is available in a number of commercial preparations and in illicit distillations of alcohol (moonshine), is metabolized to formaldehyde and then to formic acid.9 Formic acid is extremely toxic to the retina and leads to blindness, coma, and death. Ethylene glycol, common in antifreeze, gets metabolized to glycolate and oxalate, which precipitate in the kidney to cause tubular injury and obstruction.2 Treatment of these toxic ingestions involves aggressive hydration to maximize renal clearance and use of fomepizole, a competitive inhibitor of alcohol dehydrogenase. It is recommended that fomepizole be administered if any of the below criteria are met:10,11
Documented recent history of ingesting methanol or ethylene glycol and serum osmolal gap more than 10
Strong clinical suspicion of methanol or ethylene glycol poisoning with 2 of the following:
Arterial pH less than 7.3
Serum bicarbonate less than 20 mEq/L
Osmol gap more than 10
Urinary oxalate crystals present
Hemodialysis may be required in cases of severe ingestions, acidemia, or renal failure.10,11 It should also be noted that patients with methanol ingestion should be treated with folic acid (50 mg every 6 hours), as it assists with metabolism of formic acid to CO2 and water.12
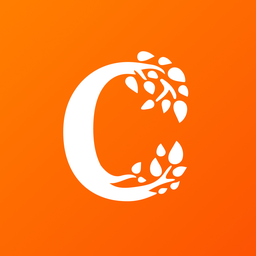
Full access? Get Clinical Tree
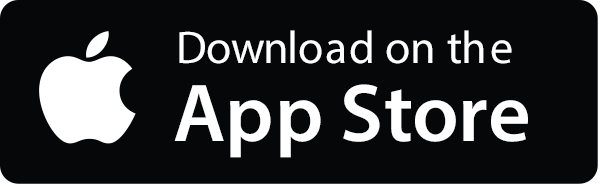
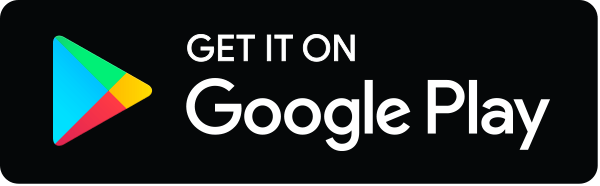