In the early 1900s, Sir Charles Sherrington designated high-intensity stimuli that signaled a potential injury to the body as being nociceptive in character. The application of such stimuli to the body produces a syndrome that includes withdrawal of the affected body part, signs of autonomic activation, and a complex set of behavioral responses that in intact animals include agitation and vocalization. In humans, an unconditioned high-intensity stimulus evokes discrete sensations that have the assigned attribute of being painful (e.g., the sensation initiated by picking up a very hot cup of coffee). The reported sensation is referred to the site of stimulation, and the magnitude of the report or response varies with the intensity of the stimulus. Termination of the stimulus before injury results in cessation of the sensation. Accordingly, nociception is a descriptor that refers to the physiologic response generated by such high-intensity, potentially tissue-injuring stimuli, whereas pain represents the interpretation of that event as a sensation with highly aversive properties. Such stimuli have powerful motivating effects that can support the generation of escape behavior when cued by innocuous stimuli that have previously been paired with a nociceptive stimulus. Thus, a strong shock evokes the nociceptive state (e.g., hypertension). A light paired with that shock would soon become conditionally associated with the shock such that in a short time, it alone can initiate the same profile of nociception.
Should the stimulus be of sufficient magnitude to result in local injury (tissue disruption, plasma extravasation), a pain sensation will persist after removal of the stimulus, and the injury will be accompanied by increased sensitivity to subsequent stimuli applied to the injury site (primary hyperalgesia) and enhanced sensitivity to stimuli applied at sites adjacent to the injury site (e.g., secondary hyperalgesia). Again, as mentioned, these states of enhanced sensation indicate that the non–tissue-injuring stimulus now acquires an aversive property (e.g., warm water on a sunburn).
As an overview, these effects of a high-intensity, tissue-injuring stimulus reflect an initial activation of the primary afferents that project to the dorsal horn of the spinal cord, from which transmitters are released that activate a complex dorsal horn circuitry. Under normal circumstances these spinal neurons respond maximally to input arising from the root projecting to the spinal segments in which the cell lies. However, even though these afferents primarily activate these homosegmental neurons, they also send collaterals rostrally and caudally up to several spinal segments away, where they make synaptic contact with neurons in adjacent segments (heterosegmental). These distal neurons are less efficiently activated than the homosegmental cells (and may not be activated sufficiently to generate an action potential), but together these homosegmental and heterosegmental cells form the real or potential dimension of the dermatome of that spinal segment. As will be shown later, if the excitability of these higher-order spinal heterosegmental neurons is increased, cells that were not activated by a given input now become depolarized, and the size of the dermatome of a given segment will be increased. These dorsal horn neurons then project by long tracts in the ventrolateral quadrant either (1) directly to diencephalic sites (e.g., thalamus, hypothalamus) or (2) indirectly through an intermediate synapse on neurons in the medulla, pons, or mesencephalon, which then project to a variety of diencephalic and limbic forebrain sites. The anatomic details of these pathways have previously been discussed (see Chapter 8 ).
In general, the processes leading to a pain state secondary to a high-intensity peripheral stimulus reflect the frequency of traffic that appears in these spinofugal pathways. Activity in the spinofugal systems is primarily dependent on the intensity of the afferent stimulus, but as will be made evident, a variety of systems serve to increase the gain of the spinal input-output function and to depress this gain. In the first case, we would anticipate that there would be an increased pain sensation associated with any given stimulus, whereas in the second case, the pain sensation produced by a given stimulus would be diminished. This dynamic property of the input-output systems represents an important characteristic of systems that process nociceptive stimuli. In the following sections we wish to consider the transmitter pharmacology that defines these synaptic linkages.
Primary Afferent Signaling
Acute Stimulation
The systems underlying this acute psychophysical experience begin with the primary sensory neuron, the afferent fiber. It possesses several intrinsic attributes:
- 1.
In the absence of a stimulus, most primary afferents show little if any ongoing activity.
- 2.
Activity in these afferents is initiated by a variety of physical or chemical stimuli, which respectively activate specific populations of primary afferents.
- 3.
The nature of the sensory information encoded by a given primary afferent is dependent on the properties of the transduction channels or receptors that are expressed on the terminals of that sensory axon.
- 4.
The frequency of firing in these afferents generated by a particular stimulus varies in a monotonic fashion with the intensity of the stimulus (e.g., temperature for a thermal-sensitive afferent). Thus, the specific population of primary afferents activated and the frequency of discharge encode the intensity of the message.
- 5.
Sensory afferents are morphologically described as Aβ, which are large, myelinated, fast-conducting axons that are typically activated by low-intensity mechanical stimuli; Aδ, smaller, myelinated, fast-conducting afferents with subpopulations that preferentially respond to thermal or mechanical, low- or high-threshold stimuli; and C, small, unmyelinated, very slowly conducting afferents that are activated by high-intensity thermal or mechanical stimuli (or both). A myelinated afferent axon typically displays specialized terminals that are particularly sensitive to mechanical distortion, which leads to a local increase in sodium current that depolarizes the axon. Unmyelinated afferent axons typically show “free nerve endings”; that is, they display no evident morphologic specialization. However, these terminals express a variety of specific transducer channels that are sensitive to specific stimuli and serve to increasingly depolarize the afferent terminal as stimulus intensity rises. When activated by the appropriate stimulus, these channels in turn activate voltage-sensitive sodium (Na v ) channels that pass Na + and initiate action potentials. As indicated in Figure 9.1 , some of these channels that transduce a physical stimulus may also be activated by a variety of chemicals. In this case, these chemicals produce a sensation that reflects the physical stimulus that is transduced by that channel (e.g., the transient receptor potential vanilloid-1 [TRPV1] receptor is activated by capsaicin, which produces a painful burning sensation, whereas menthol activates the TRPM8 cold receptor and initiates the sensation of a low temperature).
Figure 9.1
Transducer channels on a small afferent terminal. Optimal stimulus intensities for activating channels and various chemicals that also activate these channels are indicated. Different terminals express different combinations of channels, which would define the response properties of that afferent. Channel activation leads to activation of voltage-sensitive sodium (Na v ) channels. The Na v 1.8 subtype of channels is frequently present only in unmyelinated axons (C fibers). ASIC, acid-sensing ion channel; TRP, transient receptor potential.
Tissue-Injuring Stimuli
High-intensity stimuli may result in local tissue injury. Such injury will lead to cellular disruption, injury to local vascular integrity and subsequent plasma extravasation, and migration of inflammatory cells such as macrophages and neutrophils. These events give rise to the release of a variety of active factors ( Box 9.1 ). These products act through eponymous receptors that are present on the terminals of many unmyelinated axons. Activation of these receptors serves to initiate two events: (1) depolarization of the terminal leading to discharge of the afferent, with the frequency of activation being dependent on concentration, and (2) activation of intraterminal processes (phosphorylation of local membrane channels), which sensitizes the terminal such that the degree of depolarization for a given stimulus is enhanced. Such effects yield “spontaneous afferent activity” and an enhanced response to a second stimulus applied to the site of injury. These changes in the milieu of the peripheral terminal occur secondary to tissue damage and the accompanying extravasation of plasma as a result of increased permeability of the capillary wall (see Box 9.1 ). These events are responsible for the “triple response”: reddening at the site of the stimulus (reflecting local arterial dilation), local edema (increased capillary permeability), and a regional reduction in the magnitude of the stimulus required to elicit a pain response (i.e., hyperalgesia).

The mediators listed below are released by injury from macrophages, mast cells, and blood vessels. These mediators evoke spontaneous activity in otherwise silent C fibers and lower the threshold for activation in response to a ramped thermal stimulus (right: 1 vs. 2).
- 1.
Amines. Histamine (mast cells, basophils, and platelets) and serotonin (mast cells and platelets) are released by a variety of stimuli, including mechanical trauma, heat, radiation, and certain products of tissue damage.
- 2.
Kinin. Bradykinin is synthesized by a cascade triggered via activation of factor XII by agents such as kallikrein and trypsin and by physical trauma. It acts through specific bradykinin receptors (B1/B2).
- 3.
Lipidic acids. Tissue injury activates a variety of widely distributed phospholipases that free arachidonic acid (AA). AA is a substrate for a large family of enzymes, such as cyclooxygenase, to synthesize lipid mediators, including prostaglandin E 2 , prostacyclin, and thromboxane A 2 , all of which can facilitate the excitability of C fibers through specific membrane receptors (EP-r, IPR, Tx-R, respectively).
- 4.
Cytokines. Cytokines such as tumor necrosis factor-α and interleukins such as IL-1β are released by inflammatory cells (macrophages) and sensitize C fibers through eponymous binding sites.
- 5.
Proteinases. Thrombin or trypsin is released from inflammatory cells and activates specific receptors (proteinase-activated receptors).
- 6.
Neurotrophic factors. Nerve growth factor (NGF) will activate primary afferent terminals by binding to TrkA tyrosine kinase. NGF is released from fibroblasts and mast cells by injury and inflammation.
- 7.
[H]/[K]. Elevated H + (low pH) and high K + are found in injured tissue. A variety of channels present on C fibers (e.g., transient receptor potential vanilloid-1 [TRPV1]/acid-sensing ion channels [ASICs]) are activated by H + . Acid pH potentiates terminal activation by noxious heat and other chemical mediators.
- 8.
Primary afferent peptides. Calcitonin gene–related peptide and substance P are found in and released from the peripheral terminals of C fibers. These peptides produce vasodilation, plasma extravasation, and degranulation of mast cells through their respective receptors. This leads to local reddening and swelling in skin innervated by the stimulated sensory nerve.
Nerve Injury as a Stimulus
Following nerve injury arising from a variety of insults, the organisms will frequently display the development of a variety of highly aversive “spontaneous” sensations over time. These sensations are believed to arise in part from afferent traffic. Sensory axons typically display little spontaneous activity in the absence of a stimulus. This is particularly true for small, high-threshold afferents. However, after a chemical, immune, or mechanical injury to the nerve, afferent axons exhibit (1) an initial burst of afferent firing, (2) electrical silence for an interval of hours to days, and (3) the appearance over hours to days of “spontaneous “ bursting activity in both myelinated and unmyelinated axons. This ongoing activity reflects the initial dying back of the injured axon (retrograde chromatolysis) and then initiation of sprouting. Collections of these sprouts form neuromas. Recording from the afferent axon indicates that the ongoing activity originates after an interval of days to weeks from the lesioned site (neuroma) and from the dorsal root ganglion (DRG) of the injured nerve.
Several lines of evidence support the assertion that the ectopic activity in the afferent arising from the neuroma or the DRG of the injured axon is in part responsible for the observed pain behavior: (1) the onset of ectopic activity in the neuroma or DRG and the onset of pain behavior have parallel time courses, (2) pain behavior can be blocked by the application of tetrodotoxin (TTX) or local anesthetic to the neuroma or DRG, (3) dorsal rhizotomy will transiently reverse the pain behavior, and (4) irritants applied to DRG will initiate activity and yield pain behavior. A number of changes occur that can lead to prominent alterations in ongoing afferent activity.
Altered Channel Expression
A variety of channels in the sensory afferent can modulate excitability. Two major classes are the sodium channel, which carries the primary current for axonal depolarization, and a number of potassium channels. Activation of such potassium channels can reduce axon excitability. Clearly, upregulation of sodium channels or downregulation of potassium channels would have the net effect of increasing axon excitability.
Sodium Channels
A large increase in the expression of sodium channels in neuromas and DRGs occurs after nerve injury. Several sodium channel variants exist in primary afferent neurons, including subtypes designated as Na v 1.6, Na v 1.7, Na v 1.8, and Na v 1.9. Those designated as being resistant to the sodium channel blocker TTX, Na v 1.8 and Na v 1.9, are found primarily in small DRG cells (C fibers). These channels mediate slowly activating and slowly inactivating sodium currents. The importance of some of these variants in nerve injury pain states is suggested by knock-down studies wherein reduction of, for example, Na v 1.8 has no effect on baseline pain thresholds but reverses nerve injury–evoked pain states in animal models. In humans and animal models, systemic lidocaine at plasma concentrations that block ectopic activity has the ability to attenuate the hyperpathic state observed after nerve injury, thus confirming the importance of sodium channels in the post–nerve injury pain state. In humans, mutations in one sodium channel (Na v 1.7) can cause extremely painful conditions . Conversely loss-of-function mutations lead to a prominent insensitivity to pain generated by tissue-injuring stimuli. Conversely, other mutations can result in a “gain of function,” and this is correlated with syndromes such as erythromelalgia, which is characterized by severe episodic pain.
Potassium Channels
Following nerve injury, potassium currents have been shown to be reduced, thus suggesting downregulation of these channels. Potassium channel blockers increase ectopic firing after peripheral nerve injury.
Changes in the Chemical Sensitivity of Neuromas and Dorsal Root Ganglia
Sprouted terminals of an injured axon display sensitivity to a number of humoral factors, including prostanoids, catecholamines, and cytokines such as tumor necrosis factor (TNF). Several examples of how this sensitivity is related to the appearance of ongoing activity will be noted.
Cytokines
After nerve injury, release of a variety of cytokines, particularly TNF, is noted from various local inflammatory cells. These cytokines directly activate the nerve and neuroma through eponymous receptors that become expressed in the membrane after the nerve injury. The mechanisms of the TNF interaction are multiple and complicated. Acutely, TNF decreases potassium conductance in neurons, whereas longer-term effects may be initiated through activation of a variety of kinases (mitogen-activated protein kinases [MAPKs]). Behaviorally, application of TNF to the nerve results in hyperalgesia, and systemic delivery of TNF-binding protein reduces free TNF and decreases pain behavior in animals with neuropathic pain.
Catecholamines
Following nerve injury, postganglionic sympathetic efferents sprout into the injury site. In response to the release of nerve growth factors from local Schwann cells and inflammatory cells, these postganglionic terminals locally release catecholamines. Physiologic studies have shown that following nerve injury, stimulation of postganglionic axons will excite the injured axon and the DRG of the injured axon and that such activation is blocked by α-adrenergic antagonism. After nerve injury, upregulation of the expression of α 1 -adrenergic receptors has been demonstrated. Accordingly, increased catecholamine concentrations in the vicinity of the DRG or the injured neuroma can translate into enhanced activity.
Prostaglandin Receptors
Prostanoids are released by inflammatory cells secondary to tissue injury. They can enhance the opening of TTX-insensitive sodium channels by acting through eponymous receptors on the afferent terminal. TTX-insensitive channels are typically found on small unmyelinated axons, which emphasizes the association of these observations with axons carrying information having “nociceptive” content.
These events are believed to contribute to the development of “spontaneous” afferent traffic after peripheral nerve injury.
The First-Order Synapse
Primary afferents enter the spinal dorsal horn. As reviewed elsewhere, large afferents (Aβ) terminate deep to Rexed lamina III of the dorsal horn. Aδ afferent fibers terminate both superficially and in deeper laminae, whereas C fibers generally terminate superficially in laminae I and II (marginal layer and substantia gelatinosa).
Primary Afferent Transmitters
It has classically been appreciated that primary afferent input results in a postsynaptic excitatory event, thus emphasizing that primary afferent transmitters are uniformly excitatory. As a general statement, it appears that the principal primary afferent neurotransmitter evoking acute excitation is glutamate. It is contained in the synaptic vesicles of most spinal afferent terminals, and its synthetic enzymes have been identified in virtually every primary afferent DRG cell body, regardless of size or state of myelination. These acute effects are mediated by the α-amino-3-hydroxy-5-methyl-4-isoxazolepropionate (AMPA)-type glutamate ionophore present on the second-order neuron. This receptor produces a robust, but short-lasting depolarization of the postsynaptic membrane by increasing sodium conductance ( Table 9.1 ).
In addition to glutamate, populations of primary afferents may contain and release a number of neuropeptides, notably substance P (SP) and calcitonin gene–related peptide (CGRP), and even certain growth factors such as brain-derived neurotrophic factor. Given the complexity of the coding, it is likely that nociceptive information is processed by a variety of transmitters. The transmitters in these small high-threshold afferents display several general properties:
- 1.
Consistent with their location in small afferent terminals, high levels of peptides are present in laminae I and II, and these levels are reduced by rhizotomy or ganglionectomy or by treatment with the small afferent neurotoxin capsaicin. The sensitivity of these afferent to capsaicin indicates that one important characteristic of many (but not all C fibers) is expression of the TRPV1 (capsaicin) receptor. A second population of C fibers that do not express TRPV1 typically express a second marker (isolectin B4 [IB4]). These IB4-positive afferents characteristically project to the deeper layers of the dorsal horn and do not express neuropeptides.
- 2.
Glutamate and many peptides are co-contained and co-released (e.g., glutamate, SP, and CGRP in the same C-fiber terminal).
- 3.
Release is dependent on the opening of voltage-sensitive calcium channels, and the magnitude of release is proportional to stimulus frequency.
- 4.
Iontophoretic application of glutamate and the peptides found in primary afferents onto the dorsal horn will produce postsynaptic excitation. Amino acids produce a rapid, short-lasting depolarization, whereas the peptides produce a delayed and long-lasting discharge (see Table 9.1 ).
Regulation of Dorsal Horn Excitability
As noted in the introduction, the intensity of the painful stimulus is encoded in the projection to higher centers by the frequency of the output function. Accordingly, factors that enhance the excitability of spinal afferent terminals (leading to increased release of transmitter) or the excitability of the dorsal horn projection neuron will increase the apparent magnitude of a given stimulus, whereas factors that diminish the excitability of the primary afferent or the projection neuron will lead to a reduction in the apparent stimulus intensity. In the following section we will consider substrates that respectively enhance and diminish the response of the dorsal horn to a given stimulus.
Facilitation of Dorsal Horn Excitability
Persistent small (C fiber), but not large (A fiber) afferent activation of lamina I (marginal cell) and lamina V (wide dynamic range [WDR]), as occurs with tissue injury and inflammation, has been shown to (1) enhance the response to subsequent dorsal horn input and (2) increase the receptive field of the activated neurons. Thus, the conditioning afferent input increases the receptive field size of the neurons such that afferent input from dermatomal areas that previously did not activate the given neuron now evokes a prominent response. Moreover, low-threshold tactile stimulation also becomes increasingly effective in driving these neurons. This phenomenon, first described by Lorne Mendell and Patrick Wall in the mid-1960s, is broadly referred to as “wind-up.” These physiologic effects reflect events that are referred to as central or, more specifically, spinal sensitization and are believed to underlie the psychophysical correlates of tissue injury wherein tissue injury leads to hyperalgesia and secondary hyperpathia (e.g., increased sensitivity to stimuli applied outside the area of injury). The relevance of this small-afferent–evoked facilitation in humans has been emphasized by psychophysical studies in human subjects. Here, local activation of C fibers by the intradermal injection of capsaicin leads to an initial pain report followed for an extended period by a surrounding region showing enhanced mechanical and thermal sensitivity. This effect is blocked by a transient local anesthetic block of the nerves innervating the capsaicin-injected area. These observations emphasize that ongoing small-afferent input can initiate central sensitization of pain processing.
Based on the above commentary, a decrease in C-fiber–evoked excitation in the dorsal horn produced by a reduction in the release of small-afferent transmitter or block of the postsynaptic receptor (e.g., AMPA for glutamate) will diminish the magnitude of the afferent drive and, accordingly, diminish the facilitated processing evoked by protracted small-afferent input. The facilitated state, however, reflects more than the repetitive activation of a simple excitatory system.
Glutamate Receptors and Spinal Facilitation
The first real demonstration of the unique pharmacology of the facilitated state was achieved by showing that spinal wind-up was prevented by the spinal delivery of antagonists of the N -methyl- d -aspartate (NMDA) receptor, a glutamate ionophore composed of a number of subunits that is potently excitatory. When activated, it passes large amounts of Ca 2+ and Na + current. An important observation is that NMDA antagonists have no effect on acute evoked activity but reduce the wind-up induced by repetitive C-fiber stimulation. Correspondingly, behavioral studies have revealed that such drugs have no effect on behavior evoked by an acute noxious stimulus (e.g., acute thermal escape) but do reduce the hyperalgesia observed after tissue injury and inflammation.
The absence of an effect of NMDA antagonism on acute afferent-evoked activation or the pain state reflects an important property of this receptor. At resting membrane potential the NMDA receptor displays a block of the channel by Mg 2+ . Occupancy of the NMDA receptor by glutamate will not activate the ionophore in the presence of Mg 2+ . With ongoing depolarization of the membrane (as produced during repetitive stimulation) secondary to the activation of AMPA and SP receptors, the Mg 2+ block is removed. If several allosteric binding sites on the NMDA ionophore are occupied (glycine/polyamine sites), glutamate may now activate the NMDA channel and permit passage of Ca 2+ and Na + current. This opening thus serves to further depolarize the membrane and, importantly, to increase intracellular Ca 2+ , which serves to initiate downstream components of the excitatory and facilitatory cascade (see below).
It has become increasingly apparent that although AMPA mediates an acute depolarization reflecting an increase in Na + influx, in the presence of repetitive stimulation, Ca 2+ permeability develops in the AMPA channels (e.g., calcium-permeable AMPA channels with a distinct antagonist pharmacology).
Downstream Cascades
Primary afferent C fibers release peptides (e.g., SP, CGRP), purines (adenosine triphosphate [ATP]), and excitatory amino acid (glutamate) products. These peptides and excitatory amino acids induce excitation in second-order neurons. As noted for glutamate, direct monosynaptic excitation is mediated by AMPA receptors (i.e., acute primary afferent excitation of WDR neurons is not mediated by the NMDA or neurokinin 1 [NK1] receptor). This initial activation leads to enablement of the NMDA ionophore (and the generation of Ca 2+ -permeable AMPA channels), which in turn initiates a number of complex cascades that reflect processes that serve to “sensitize” the postsynaptic dorsal horn neuron. Several primary examples of such facilitatory cascades initiated by repetitive small-afferent input are presented in the following text.
Local Neuronal Circuits
NMDA Receptors
The best example of a cascade initiated by small-afferent input is activation of the NMDA receptor. As noted above, with ongoing membrane depolarization, the NMDA ionophore loses its Mg 2+ block, which allows the large increase in intracellular Ca 2+ .
AMPA Receptors
Although the AMPA receptor is largely considered to be an acutely activated ionophore that allows the primary passage of sodium, there are structural variants on the AMPA receptor that allow it to pass calcium. These “calcium-permeable” AMPA receptors are believed to participate along with the NMDA receptor in various aspects of spinal facilitation.
Neurokinin 1 Receptors
NK1 receptors are G protein–coupled receptors that are activated by the SP released from small primary afferents. Activation of this receptor leads to prolonged depolarization and mobilization of intracellular calcium. Spinal delivery of agents that block the NK1 site for SP can diminish wind-up and significantly reduce the second-phase response to intradermal formalin injection.
Prostaglandin Cascade
The increase in intracellular calcium serves to promote the transmigration of a variety of phospholipases to the membrane, where they then serve to cleave arachidonic acid. Arachidonic acid serves as a substrate for cyclooxygenase (COX) to produce a number of prostanoids. All these enzymes have been found to be constitutively expressed in spinal neurons and non-neuronal cells. This cascade leads to the release of a number of prostaglandins that act on a number of eponymous receptors that are located presynaptically on the primary afferent terminal and postsynaptically on second-order neurons. The presynaptic effect has been shown to enhance the opening of voltage-sensitive calcium channels, which serves to mediate vesicular mobilization and release of transmitter. A postsynaptic action that attenuates the activation of inhibitory glycine receptors has been demonstrated. This leads to loss of the regulatory inhibition that otherwise limits postsynaptic activation. In turn, this results in an enhanced response of the second-order (projection) neuron to a given afferent input ( Fig. 9.2 ).
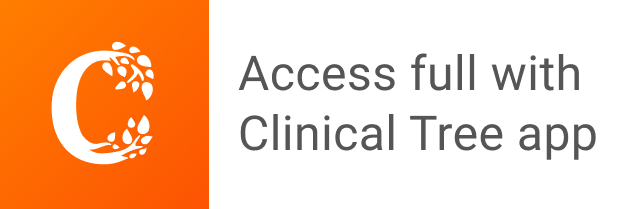