Introduction
Many therapies and interventions are not based on proven benefit, but on anecdotal evidence, expert opinion, and tradition. This is especially true for oxygen therapy, which is usually not questioned and has been used for more than 100 years. However, such a practice may be harmful when it is not truly necessary [1–5]; some of the available evidence will be provided in this chapter.
Oxygen is one of the most frequent drugs administered during critical care and during the pre-, intra-, and postoperative periods, and its administration and management has been fraught with problems, misunderstandings, and errors. We have all been taught about the many causes of hypoxemia and of the potential significant damage secondary to hypoxia. This is well taught in medical and nursing schools and throughout residency. Based on this education, no healthcare provider induces or tolerates hypoxemia in any patient. Regrettably, in many places there is still a lack of education about the potential damaging effects of hyperoxemia and hyperoxia. Additionally, and very differently from hypoxemia and hypoxia, the only reason that hyperoxemia can occur in a human being is us, the healthcare providers. We cause hyperoxemia and hyperoxia in the laudable effort to prevent or treat hypoxemia and when supplementary O2 is provided unnecessarily. Even though we still do not know the perfect way to manage this potent drug, we have learned during the last decade that there are many things we should not continue to do as we have been doing [2–10].
This chapter reviews several aspects of oxygenation and oxygen management. The focus is more on hyperoxemia and hyperoxia, since hypoxia and hypoxemia are better known and well covered in many other places. With this in mind, this chapter describes the following in its different sections: (1) physiology of oxygenation; (2) oxidative stress and oxidant injury; (3) oxygen as a health hazard; (4) assessment of oxygen in blood; and (5) targeting of SpO2 and recommendations for clinical practice.
Physiology of Oxygenation
Atmospheric pressure (Patm) is about 765 mmHg at sea level. We all know that in room air the inspired fraction of O2 (FiO2) is 0.21; the rest (0.79) is nitrogen, regardless of the altitude above sea level. That is to say that 21 percent of the Patm is the partial pressure of inspired O2 (PiO2). If we are interested in knowing the PiO2 of dry gas we have to subtract the estimated water vapor pressure (PH2O), which is variable and frequently estimated at about 47–50 mmHg. Therefore, the PiO2 in room air at sea level (Patm of about 765 mmHg) is about 150 mmHg (Patm – PH2O × 21 / 100), varying also with ambient temperature. Of course, relative ambient humidity (water vapor pressure) is also variable. So the partial pressure of O2 that reaches the upper and lower airway is about 150 mmHg, and this will reach the alveoli. Here there is another gas, CO2, at an alveolar partial pressure (PACO2) of about 40 mmHg. Therefore, “dry” alveolar PO2 (PAO2) in room air is about 110 mmHg (PiO2 – PACO2). This is in few and simple words the alveolar gas equation; the known formulae are given below.
The PAO2 then has to reach pulmonary capillaries and veins, left atrium, left ventricle, and systemic circulation. In normal healthy children and adults the PaO2 is about 100 mmHg. In the neonatal period, due to intra- and extrapulmonary shunting, PaO2 is usually 45–75 mmHg and rarely >80 mmHg.
Once in systemic circulation, oxygen has to be transported by hemoglobin (Hb) and circulated in the blood to reach tissues for adequate tissue oxygenation. However, tissue oxygenation is complex and multiple factors are related to achieving this process satisfactorily (Table 7.1).
Table 7.1 Factors related to tissue oxygenation
PaO2 (mmHg) Hb concentration (anemia) and quality (Hb F; Hb A; dyshemoglobins) Hb–O2 relationship (saturation in percent) Oxygen content (CaO2 in ml O2/dl) [(SaO2 × Hb × 1.34) +.003 ml O2/mmHg PaO2]. Cardiac output (heart rate; stroke volume; minute volume) Peripheral vascular resistance and systemic blood flow Pulmonary vascular resistance and blood flow | Hematocrit (hyperviscosity) pH CO2 local and systemic Temperature Glycemia O2 delivery O2 consumption PaO2 local (4–20 mmHg) Distance Microcirculation |
Of all this, we frequently and easily measure PaO2, but this is probably among the least important factors related to tissue oxygenation. Thus, there could be a low PaO2 in blood (hypoxemia) with adequate tissue oxygenation (normoxia); furthermore, there could be normoxemia and even hyperoxemia but not sufficient oxygen in the tissue (hypoxia). A normal (or high) PaO2 with tissue hypoxia generally occurs in one of several ways: (1) anemia; (2) altered affinity of hemoglobin for binding oxygen (for example in cases of methemoglobinemia or when the Hb–O2 curve shifts markedly to the right); (3) poor perfusion (altered cardiac output and vascular resistance); (4) decreased oxygen delivery; or (5) altered microcirculation. In summary, to remain viable tissues require a certain amount of oxygen per minute, a need met by oxygen content (CaO2) and delivery, and not oxygen pressure.
Oxygen has to be bound to Hb to be transported to the tissues; dissolved oxygen has extremely little participation in this process. In normal conditions, the normal Hb–O2 curve is sigmoid and maintains a predictable relation between PaO2 and arterial saturation (SaO2). One gram of Hb can carry about 1.34 ml of O2 when fully saturated, a concept known as maximum oxygen carrying capacity. To make the necessary connection between PaO2 and O2 content requires applying the CaO2 equation (described in Table 7.1). As shown, SaO2 and Hb are key factors, while a very small quantity of oxygen is carried dissolved in plasma (0.003 ml O2/mmHg PaO2; which is to say only 0.3 ml O2/dl plasma when PaO2 is 100 mmHg). The following examples illustrate these concepts, using values of Hb that exist in neonates:
With SaO2 of 100 percent and Hb of 20 g dl–1: CaO2 is 26.8 ml dl–1 (+0.003 ml O2/mmHg PaO2)
With SaO2 of 100 percent and Hb of 8 g dl–1: CaO2 is 10.72 ml dl–1 (+0.003 ml O2/mmHg PaO2)
If desaturation occurs in the first case, CaO2 is still usually much higher than in the second one:
SaO2 of 90 percent and Hb of 20 g dl–1: CaO2 is 24.12 ml dl–1. Anemia, even with normal SaO2, can therefore lead to hypoxia.
The most common method to try to clinically assess oxygenation is the noninvasive continuous measurement of arterial saturation by pulse oximetry (SpO2), currently considered a “fifth vital sign.” However, the pulse oximeter tells us very little about tissue oxygenation. In many clinical situations and practices, methods to determine cardiac output are infrequently available or not available at all. More so, in most situations peripheral vascular resistance, O2 delivery and consumption, microcirculatory changes, and other factors cannot be assessed in critically ill infants and function of precapillary sphincters and of postcapillary venules is ignored in clinical care.
In summary, tissue oxygenation is a complex process involving many factors. Due to the issues discussed here and other factors, we need to handle O2 administration with extreme care to preserve tissue oxygenation and prevent and treat hypoxia adequately, while at the same time always trying to avoid causing damage by inducing hyperoxemia, hyperoxia, oxidative stress, and oxidant damage.
Oxidative Stress and Oxidant Injury
We all know that oxidation can be destructive. With iron and other metals O2 creates a slow-burning process, which results in the brittle brown substance we call rust. The interaction between O2 molecules and all the different substances they may contact can lead to oxidation. Chemically, oxidation is the loss of at least one electron when two or more substances interact.
Various environmental stresses lead to excessive production of reactive oxygen species (ROS), causing progressive oxidative damage and ultimately cell death. It is the imbalance between the systemic manifestation of ROS and a biological system’s ability to readily detoxify the reactive intermediates or to repair the resulting oxidative damage. Thus, oxidative damage to the tissues depends on the delicate equilibrium between ROS production and their scavenging.
Mechanisms of DNA oxidation had been well described and summarized by the end of the previous century [11,12]. Every day there is about 1012 molecules of O2 that enter each and every one of our cells, and there are an estimated 37.2 trillion cells in the adult human body! [13]. When 1/200 O2 molecules are not balanced by antioxidants and act freely, our DNA starts to get affected; 1/100 affects our proteins [11,12]. In our daily lives, in normoxia, we are all subject to oxidant stress and oxidative damage. This is a well-known reason that our cells age in normoxia. The groups of genes that are more upregulated in aging are the response genes to oxidative stress and DNA damage. The repressor element 1-silencing transcription factor (REST) is a universal feature of normal aging in human cortical and hippocampal neurons. REST potently protects neurons from oxidative stress and amyloid beta-protein toxicity; conditional deletion of REST in the mouse brain leads to more oxidative stress and age-related neurodegeneration [14]. This obviously would not happen in hypoxia but, since we all know of the potentially serious side-effects of tissue hypoxia, we “tradeoff” accepting the perils of oxidant stress and live in normoxia. However, as care providers we must do everything possible to avoid inducing worse oxidative damage by producing and accepting hyperoxia when we care for patients.
Highly reactive ROS are produced by stepwise reduction of molecular oxygen (O2) by high-energy exposure or electron-transfer reactions. Reactive oxygen species are summarized in Table 7.2. Some ROS are important as they act as cellular messengers in redox signaling, but excess or imbalance with antioxidants generates stress and disruptions in normal mechanisms of cellular signaling. Peroxides and free radicals can damage all components of the cell (proteins, lipids, and DNA).
Table 7.2 Radical oxygen species
Free radicals | Nonradical molecules |
---|---|
• Superoxide anion (O2•−) • Hydroxyl radical (•OH) 2 O2•−+2H+→ H2O2 + O2 2 O2•− + 2H+ → •OH + •OH | • Hydrogen peroxide (H2O2) • Singlet oxygen (1O2) • Others |
The antioxidant system that protects against significant oxidative damage is well developed in adults, but there is individual variability in its functioning. The best-known antioxidant defenses are summarized in Table 7.3. This system, however, is poorly developed or markedly impaired in newborns and infants, and is even more defective in preterm infants. This is one reason why hyperoxia and oxidative damage are more serious during development.
Table 7.3 Summary of antioxidant defenses
Enzymatic | Nonenzymic/dietary |
---|---|
Superoxide dismutase (SOD) Catalase (CAT) Guaiacol peroxidase (GPX) Ascorbate-glutathione (AsA-GSH) Ascorbate peroxidase (APX)]. Monodehydroascorbate reductase (MDHAR) Dehydroascorbate reductase (DHAR) | Ascorbate (AsA) Glutathione (GSH) Carotenoids Tocopherols (vitamin E) Coenzyme Q10 Vitamin C Phenolics |
Acute oxygen therapy may have acute undesired effects such as an impact on respiratory control and on chemo- and baroreceptors, raised blood pressure and decreased cardiac index, heart rate, cardiac oxygen consumption, and blood flow in the cerebral and renal beds. It may also lower capillary density and redistribute blood in the microcirculation. Table 7.4 summarizes adverse conditions and damage associated with oxidant stress. Using excess oxygen for the treatment of nonhypoxemic patients is definitely a health hazard.
Table 7.4 Conditions associated to hyperoxia, oxidative stress, and damage
Aging DNA impact – cancer Central nervous system • Parkinson’s disease • Alzheimer’s disease • Neuronal degeneration • Autism • Altered blood flow Cardiovascular • Atherosclerosis • Heart failure • Myocardial infarction • Decreased contractility • Altered chemo- and baroreceptors Endocrine • Insulin • Glucagon Lung • Atelectasis • Alveolar collapse | Other • Fragile X syndrome • Sickle cell disease • Lichen planus • Vitiligo • Infection • Inflammation – fibrosis • Chronic fatigue syndrome • Altered renal function Perinatal: Specific to mother, fetus, and neonate • Placental defenses and genes • ROP • BPD – lung injury • Developing brain • Increased PVR • Abnormal response to iNO • Altered clock genes • Altered ocular genes • Thymus (T-cells) |
ROP: retinopathy of prematurity; BPD: bronchopulmonary dysplasia; PVR: pulmonary vascular resistance; iNO: inhaled nitric oxide.
Oxygen as a Health Hazard
A hazardous substance is one for which there is statistically significant evidence that danger to health, with acute or chronic health effects, may occur from exposure. OSHA’s definition includes any substance, chemical, or agent that is, among other things, a carcinogen, damages the lungs and eyes, is combustible, explosive or flammable, oxidizes, and is unstable and reactive such that its use may produce or release other compounds that have any of the previously mentioned characteristics. Oxygen is a very potent drug that meets these criteria and has been used in inadequate ways in many healthcare settings. We must change the way we administer supplementary O2 when a patient is not hypoxemic and stop using pure free-flowing oxygen, since 100 percent oxygen unleashes a cascade of dangerous chemicals [15]. More so, periods of fluctuating hypoxia–hyperoxia reperfusion are potentially very damaging [16–18].
Table 7.4 lists a summary of potential side-effects of O2. Important conditions related to oxygen damage in adults are described, followed by several that may occur during the perinatal and infancy periods.
Most patients with acute myocardial infarction or acute coronary syndromes (ACS) receive O2 therapy as part of their treatment, initiated most often before their first contact with a physician, but there is no evidence that this is beneficial. A survey among physicians found that 96 percent of their patients with ACS received oxygen therapy [19]. About 50 percent of all responders believed that O2 decreases mortality, but this has never been shown. In fact, considerable data suggest that O2 therapy may be detrimental in patients with both ACS and stable coronary disease when there is no hypoxia. O2 administration may constrict the coronary vessels, lower myocardial oxygen delivery, and may actually worsen ischemia. Therefore, this long-accepted but potentially harmful intervention needs to be revised, favoring use of O2 only in hypoxemic patients, and titrating its administration to individual PaO2 or SpO2 [20].
The role played by oxidatively damaged DNA in carcinogenesis has been established. Oxygen free radicals’ interaction with DNA can result in genetic changes which can lead to cancer [21]. Oxidative stress has been shown to contribute to the development of various cancer types and has recently been involved in the pathogenesis of pancreatic cancer [22]. Clinical studies on the effect of preventive antioxidants have shown surprisingly little or no effect on cancer incidence, and epidemiological trials, together with in vitro experiments, suggest that the optimal approach is not to increase intake of antioxidants but to reduce endogenous and exogenous sources of oxidative stress. One of the exogenous sources is clearly breathing supplemental O2 when it is not needed. Additionally, a randomized study evaluating recovery post-anesthesia with 80 percent vs. 21 percent O2 was interrupted by the safety review board as the incidence of infections was markedly higher in the O2 group [23].
Both the mother and neonate are exposed to oxidative stress during birth. However, lipid peroxidation levels and antioxidant capacity in umbilical venous and arterial blood immediately after delivery at over 37 weeks’ gestation are significantly different in patients delivered by planned Cesarean section (C-section) when compared to vaginal delivery [24]. The higher oxidative stress and alteration of the oxidant and antioxidant system during C-section must be added to the list of the many known risks associated with planned elective C-sections. Worse, if O2 is used unnecessarily during C-sections and labor and delivery, the potential damage caused to mothers, placenta, and fetus increases markedly [25–32].
In a random prospective study [25], supplemental oxygen (FiO2 0.4) was compared to room air (FiO2 0.21) in elective C-sections under spinal anesthesia. Total antioxidant capacity, total oxidant status, and the oxidative stress index were measured in maternal pre- and postoperative blood and in umbilical artery blood samples. Fetal and maternal oxidative stress was significantly worse with FiO2 0.4 than with room air. The authors suggested a mandate to warn about the use of supplemental oxygen during this procedure and wrote that we must “handle the sword with care.” A meta-analysis of the effect of inspired oxygen concentration and a Cochrane review, both from 2013, report significant differences in healthy pregnant women with supplemental O2 during C-section with regional anesthesia compared with no supplemental O2 [29,30]. PaO2 in mother and neonate, and free O2 radicals and ROS were all significantly higher with administration of supplemental O2. Mean difference above the values found in room air for maternal PaO2 was 141.8 mmHg, and for neonatal umbilical artery and vein PO2 were 3.3 mmHg and 5.9 mmHg respectively (p < 0.0001). The levels of the O2 free radicals malondialdehyde (MDA) and 8-isoprostane were also significantly higher (0.2 µmol L–1 and 64.3 pg ml–1, respectively, p < 0.00001).
The numerous functions of the placenta are astounding, but many are still not fully understood and the effects of hyperoxia have only recently started to be studied. Proteins of resistance to multiple drugs (glycoprotein-P) and of resistance to breast cancer (genes ABCB1 and ABCG2) are highly expressed in the placenta during the first trimester and they protect the fetus from maternally derived toxins and drugs, foreign chemical substances, and pollutants (i.e., xenobiotics). As O2 is a regulator of the resistance to multiple drugs in various tissues, the effect of oxygen on multidrug resistance was studied in explants of villi of human placentas at different times during gestation. It was found that ABCG2 mRNA increases after hours of hyperoxia. It is possible, therefore, that placental changes in O2 tension and delivery may alter resistance to drug and toxin exposure during fetal life. In a different study, increased PaO₂ reduced the cellular pro-inflammatory response to E. coli and promoted an abnormal anti-inflammatory cytokine profile in the membranes. In addition, inflammatory pathways connect hyperoxic induced intra-amniotic inflammation with fetal and neonatal tissue damage. Even though the mechanisms and molecular pathways of acute lung injury in preterm fetuses and neonates are complex, tissue injury may occur as a result of injurious O2 byproducts secondary to hyperoxia [31]. Finally, supplemental O2 has been used for intrauterine resuscitation, but it is a practice of unproven benefit and potentially harmful due to increased free radical activity [32].
In summary, maternal O2 supplementation during labor and in C-sections is potentially dangerous for mother and fetus, should be reserved only for documented maternal hypoxia, and should not be considered an indicated intervention for non-reassuring fetal status.
Neonatal and pediatric undesired effects of excess O2 are listed in Table 7.4. Harper, studying 14 healthy children aged 8–15 with functional MRI, showed that only two minutes of 100 percent O2 produces abnormal activation of the hippocampus, hypothalamus, and insula, areas that regulate blood pressure, heart rate, and response to stress [15]. Oxygen by itself also causes cell death in the developing brain [33,34], and worse long-term neurodevelopment outcomes have been associated with hyperoxia [9]. Oxygen toxicity has been identified as a contributing factor to the pathogenesis of cerebral palsy. In a large study of 1105 preterm infants [35], the risk of disabling cerebral palsy was double in those exposed to hyperoxia and eight-fold in the highest quintiles of oxygen exposure. The association of O2 excess with retinopathy of prematurity (ROP) has been known since the 1940s and better characterized more recently [5,6,8,10,36–38]; the case is the same with bronchopulmonary dysplasia (BPD) [8,39] and other conditions in which the benefits of changing practice to try to maintain SpO2 to decrease hyperoxia have been proven of benefit and are discussed later.
O2 is a pulmonary vasodilator and hypoxia induces pulmonary vasoconstriction and increased pulmonary vascular resistance. However, pure O2 produces oxidative stress in lungs and vessels, and alters pulmonary artery contractility. In animal models of persistent pulmonary hypertension of the newborn (PPHN), 100 percent O2 increases ROS in the mitochondrial matrix prior to the cytosol and can produce significant changes in critical cellular signaling pathways. Additionally, 100 percent O2 increases PDE5 activity, decreases NO-mediated cGMP-dependent vasorelaxation, and impairs the response to inhaled nitric oxide (iNO). Some of these alterations are prevented with the use of antioxidants such as superoxide dismutase and catalase, confirming the role of oxidative stress in these abnormal responses [40–42].
There has been growing interest in the impact of hyperoxia on gene alteration. Proteome changes are induced by oxidative stress in the developing brain [33,34], and two studies described a three-fold increase in the risk of leukemia and cancer in childhood with exposure to 100 percent O2 in the delivery room for three minutes or more [43,44]. In experimental animals, a few minutes of hyperoxia at birth alter whole-genome expression in the newborn eye [45]. If this were to occur during induced hyperoxia after birth of preterm infants, predisposition for ROP could be markedly increased. Hyperoxic acute lung injury is characterized by inflammation and epithelial cell death. Circadian locomotor output cycles kaput (CLOCK) genes, master regulators of circadian rhythm, integrate various antioxidant and metabolic pathways. Circadian rhythm disruption is associated with many pathological conditions and is implicated in inflammation and lung diseases. Key circadian genes like Rev-erbα, Per, and Bmal modulate metabolism and inflammation. Hyperoxia-induced lung inflammation and injury can be promoted by dysregulation of circadian rhythm by alteration of CLOCK gene expression [46,47]. For example, Rev-erbα protein is degraded in hyperoxia and its disruption exacerbates hyperoxic lung injury, which is associated with decreased cell proliferation and distorted alveolar architecture. Stabilizing lung Rev-erbα protein prevents the loss of cell proliferation. Premature neonates are often exposed to hyperoxia and photo-oxidation with phototherapy in the NICU. In neonates <750 g birth weight, aggressive phototherapy was found to increase mortality. The potential cytotoxicity and disruption of circadian gene expression altering cell homeostasis as a combined effect of O2 and phototherapy is concerning since preliminary data suggest that an alteration of circadian rhythm genes occurs in neonatal animals exposed to some hyperoxia associated with phototherapy. This cytotoxic effect can be partly reversed by stabilizing the Rev-erbα protein, which improves mitochondrial efficiency, conferring a survival advantage [47].
Oxygen should never be denied when necessary. Nonetheless, to avoid hypoxemia without inducing hyperoxemia is neither easy nor simple. Understanding oxygen toxicity provides us with new ways of interrupting pathologic sequences since the hazards described are “in our hands.” We, the healthcare providers, are the only cause for a PaO2 greater than normal in a human being of any age in a healthcare setting. We therefore have to assess O2 levels and manage O2 administration differently than we have been doing.
Assessment of Oxygen in Blood
Cyanosis is the bluish discoloration of skin and mucous membranes due to an increased concentration of reduced (not saturated) Hb. As much as this sign is important, we must recognize its pitfalls. Human beings perceive color with variability. Furthermore, illumination, race, skin thickness, and total Hb concentration impact on this clinical assessment. We all have a “blind spot” for detecting cyanosis on the face of even significant Hb desaturation, more so in cases when total Hb concentration is low. In summary, some of the patients with what appears to be cyanosis do not need supplemental O2, and in many more in whom we do not see cyanosis there is hypoxemia and a need for some evaluation and therapy. SpO2 is currently the noninvasive way to assess hypoxemia.
Clinically we assess oxygen in blood by intermittent measurements of PaO2 and/or SaO2 in arterial or venous blood and by continuous noninvasive pulse oximetry (SpO2) monitors. The pulse oximeter does not measure the same thing as arterial blood gas. SpO2 monitors measure the Hb-O2 saturation, while the arterial blood gas measures the pressure of oxygen gas dissolved in the blood (oxygen not bound to hemoglobin) and “calculates” oxygen saturation. The calculation or transformation of PaO2 to SaO2 by means of formulae or algorithms is of no value in hospitalized ill patients and in all newborns; therefore a SaO2 derived from a blood gas should not be used for clinical management. Such calculation assumes a normal adult O2 dissociation curve and O2 affinity, with normal 2,3-DPG and Hb concentration, and a normal temperature, pH, and PaCO2 for healthy adults. It also assumes that the patient has no dyshemoglobinemia and is without fetal hemoglobin (Hb F). These calculations are therefore correct only under standardized normal conditions that are usually not present in hospitalized pediatric patients with variable pH, PaCO2, and temperature, and are never found in neonates in whom, in addition, a large and variable proportion of Hb is Hb F (not A), 2,3-DPG is low and Hb concentration is variable. Therefore, continuously monitored SpO2 cannot be compared to a calculated SaO2 derived from a PaO2 from an arterial blood gas. To circumvent this problem, many healthcare settings customize the output of blood gas analyzers so they do not report SaO2. Good and modern co-oximetry is the gold standard for SaO2. Current models are called continuous wave spectrophotometers; these measure light absorbance at 100–130 µm wavelengths. However, co-oximeters are expensive, require blood sampling, and provide only intermittent, not continuous, monitoring. This is not ideal when one wants to closely monitor an unstable patient. Noninvasive pulse oximetry is used routinely to monitor SpO2 continuously. They were created to aid in the diagnosis of desaturation, but they are of little to no value by themselves to tell us about hyperoxemia. The normal SpO2 in room air (FiO2 0.21) is 95–100 percent. However, as shown in Figure 7.1, when a patient receives supplemental FiO2 (i.e., >0.21) and the SpO2 is >95 percent (i.e., “Normal”), there is a risk for the PaO2 be above normal [48] because when we breathe supplemental oxygen and the SpO2 is >95 percent the Hb–O2 relation is “lost” (Figure 7.1). When this happens it is impossible to predict PaO2 from the SpO2 digital readout. In the example in the figure, when SpO2 is 86–93 percent, PaO2 is 50–68 mmHg. However, when SpO2 is 95–100 percent, the PaO2 could be much higher than 85 mmHg. Following the concepts of the alveolar gas equation described earlier, the PaO2 could actually be as high as 200–300 mmHg, or even higher if the lungs are normal, the SpO2 is >95 percent, and the child is breathing pure oxygen (FiO2 1.0). In these cases, free dissolved O2 will be circulating and ROS will increase (Figure 7.1). Therefore, in such situations, FiO2 should be weaned.
The way these monitors function and their strengths and limitations are well-described [5], but they are beyond the scope of this chapter. In summary, SpO2 monitors function by establishing the ratio between wavelength (red and IR) and displaying the ratio as SpO2. SpO2 monitors, just like any other medical equipment, are not perfect and all have an inherent bias (equipment error). In individuals under normal conditions this bias (or “standard deviation”) could be as low as 2 percent in some monitors but as high as 5 percent in others. Therefore, it is nonsensical to argue about differences in SpO2 values of 1–2 percent. Additionally, there are many SpO2 monitors available in the market and there are more than 100 published studies that demonstrate that one monitor is not the same as another monitor. The SpO2 monitor with Signal Extraction Technology (SET®) has been demonstrated to be the one with better specificity and sensitivity, and to monitor the patient best when it is needed the most – during critical illness and periods of low perfusion and motion. Additionally, only one monitor on the market actually uses many wavelengths (not just two) and can measure Hb, methemoglobin, and carboxyhemoglobin in addition to SpO2 (Rainbow, Masimo Corporation, Irvine, CA).
Recommendations for Clinical Practice and Targeting of SpO2
The SpO2 in normal individuals in room air is 95–100 percent. However, if SpO2 is >94 percent when breathing supplemental O2, there is an abnormally elevated PaO2 in 60 percent of arterial samples [48]. With the aim of avoiding hypoxemia and at the same time preventing hyperoxemic-induced oxidant stress, when a patient breaths supplemental O2 we should target SpO2 to avoid 96–100 percent and wean the FiO2 in such cases. In all cases, SpO2 alarms must be operative when a patient is receiving supplemental O2; the low alarm should be about 1 percent below the lower limit chosen as acceptable according to the patient and diagnosis, and the high alarm 1 percent above the acceptable high limit of SpO2. We include below clinical recommendations for cardiopulmonary resuscitation, apnea, surgery and anesthesia, PPHN, BPD, and during the first weeks of life in tiny preterm infants.
In all hospital settings, including operating rooms, pre- and post-anesthesia, and birthing centers, a heater humidifier (to provide adequately conditioned inhaled gas) and a blender (to accurately measure the dose of O2) are mandatory. In addition, an adequate SpO2 monitor that can rapidly measure SpO2 even during poor perfusion is necessary to titrate the amount of O2 administered. During cardiopulmonary resuscitation, particularly when the lungs are not affected, there is no need to provide any supplemental O2, less so 100 percent O2. Such a practice increases the risk of damage by hypoxia–hyperoxia reperfusion injury. Clear physiologic evidence that in many cases there is absolutely no need to provide excess O2, with its associated risks, could be derived by the alveolar gas equation described earlier. The FiO2 provided by mouth-to-mouth resuscitation is about 17 percent due to the exhaled CO2 in the gas provided by the person providing resuscitation, and many human beings have been successfully resuscitated in this way with this FiO2. A similar situation occurs in periods of apnea (spontaneous or medically induced), where the treatment is to ventilate the alveoli (breathe) and not to give supplemental O2.
Newborns and children who require surgery are developing and many are critically ill, but the use of O2 during neonatal and pediatric anesthesia has not been well studied. However, it has been over 45 years since Van Den Brenk and Jamieson [49] showed that, in mammals, there is potentiation by anesthetics of brain damage due to breathing high-pressure oxygen and that excess oxygenation during surgery of preterm infants is associated with ROP [50]. More recently there is concern about the negative impact of sedatives like midazolam [7] and ketamine, which alters NMDA receptors [51] and enhances human neural stem cell proliferation and induces neuronal apoptosis via ROS [52]. For these and other reasons the association of hyperoxia-induced oxidative stress and anesthetic drugs should be avoided and the practice of preoxygenation must be carefully evaluated. In addition, atelectasis can occur during and after anesthesia, and the rate at which absorption atelectasis occurs can be accurately predicted by known physiological principles: High FiO2 worsens compliance and pure O2 results in reabsorption atelectasis shortly after its application [50–58]. The alveolar to venous pressure gradient must be countered by the respiratory elastic recoil to keep alveoli open, but this gradient is too high with 100 percent O2. With just three minutes of “preoxygenation,” the higher the FiO2, the greater the areas of atelectasis and the shorter the time required for collapse to occur, irrespective of the FiO2 used after induction. Alveolar recruitment maneuvers to maintain functional residual capacity (FRC) and ventilation distribution are useful and more effective if done with positive end-expiratory pressure (PEEP) of 6 cmH2O with low FiO2. When an FiO2 of 1.0 is used, there is rapid recurrence of the atelectasis, decreased FRC, and alteration of ventilation homogeneity and lung units with low VA/Q ratios become more unstable when breathing O2 [50–58]. Moreover, an FiO2 of 1.0 before extubation increases the risk for postoperative atelectasis and more O2 than necessary in the postoperative period can, paradoxically, aggravate central hypoventilation and apnea. Mental changes during oxygen therapy were described more than 65 years ago by Comroe [1]. Since then the body of knowledge showing potential adverse effects of providing unnecessary O2 has expanded exponentially, showing that excess O2 should be avoided before, during, and after anesthesia.
In congenital diaphragmatic hernia (CDH) with PPHN, SpO2 monitoring should be in the preductal territory (right hand). But if this is unavailable, in >80 percent of babies the left hand is preductal too. In the face of the abnormal vessel and alveolar development present in CDH, it is paramount to avoid any additional hypoxic pulmonary vasoconstriction; the challenge is to do this and at the same time avoid hyperoxic injury. Without any published solid evidence, this may be accomplished by aiming to maintain preductal SpO2 between 91 and 96 percent, and weaning respiratory pressures and FiO2 when SpO2 ≥96 percent. The same is applicable to PPHN of other etiologies. Another challenging condition is chronic lung disease, where hypoxia leads to cor pulmonale, bronchoconstriction, and pulmonary vasoconstriction, but where FiO2 should be titrated with the intention to maintain SpO2 between 90 and 95 percent.
The ideal and best SpO2 targets for preterm infants breathing O2 during the first weeks of life has been a matter of controversy, but has been studied in detail [5,6,8–10,36–38]. For these infants a prudent approach is to avoid SpO2 >94 percent, with an intention to treat with SpO2 ranges of 87–94 percent and low and high SpO2 alarms of 85 and 95 percent, respectively. When any dose of supplemental O2 is given in these infants, monitoring with modern SpO2 with SET technology to aim to avoid hyperoxemia and hypoxemic episodes followed by hyperoxia reperfusion is associated with decreased rates of severe ROP and does not increase mortality [5,10,38,59].
We have recently summarized what could be a safe oxygen saturation intention to treat and monitoring in preterm infants, with the aim to avoid hypoxia and hyperoxia [60,61]. In the last few years it has become clearer that oxidative stress is the unifying element in the process of perinatal programming of adult diseases. Oxidative stress may lead to cardiovascular and other diseases, but also to changes in neuro hormones and personality disorders [62,63]. Therefore, avoiding hyperoxia and induced oxidative stress can help prevent the neonatal origin of adult diseases. Regional anesthesia with supplemental oxygen is potentially harmful and unnecessary, as summarized in Cochrane reviews in 2016 [64].
In summary, cellular oxygenation is complex. Oxygen as a gas can be similar to the Janus god and Trojan Horse in Greek mythology. The former was a god of duality, two-faced and looking at opposing sides. The Trojan Horse was a present, perceived as likeable and innocuous but capable of generating serious consequences and igniting everything without anyone realizing until it was too late. Maybe our ability to clinically measure tissue oxygenation will improve in the future. In the meantime, we must do everything possible to avoid and treat hypoxemia, while at the same time be determined not to induce hyperoxemia, hyperoxia or oxidative injury.
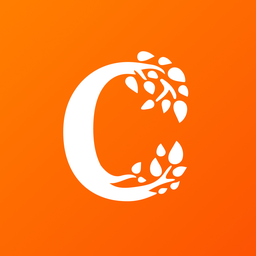
Full access? Get Clinical Tree
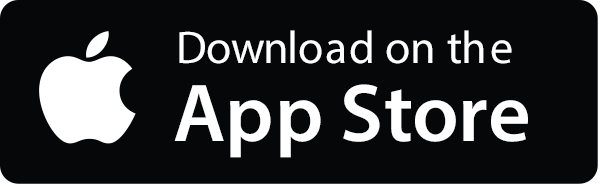
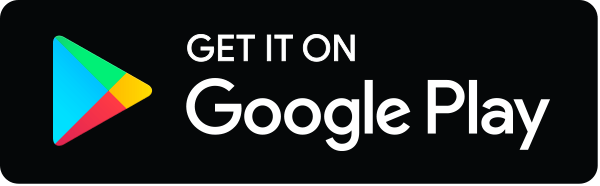