The physiologic changes that occur at birth and continue over the first few days and the first months of life are considerable and need to be appreciated in order to understand the hemodynamics of newborns.
Fetal Circulation
Fetal circulatory channels shunt blood away from the lung, such that both ventricles, in parallel, contribute to systemic oxygen delivery by pumping blood to the systemic arterial system. This parallel circulation permits normal fetal growth and development even in fetuses with cardiac malformations.
Oxygenated blood from the placenta returns to the fetus via the umbilical vein, which enters the portal venous system. The ductus venosus connects the left portal vein to the left hepatic vein at its junction with the inferior vena cava (IVC). This allows approximately 50 percent of umbilical venous blood to bypass the hepatic sinuses. The remainder of the umbilical venous flow passes through the liver and enters the IVC via the hepatic veins. Fetal IVC blood is a combination of blood from the lower fetal body, umbilical vein, and hepatic veins. The stream of blood from the ductus venosus has a higher velocity in the IVC than the stream from the lower body and hepatic veins. This higher velocity facilitates delivery of this higher oxygen content blood across the foramen ovale (FO) into the left atrium (LA) [1] (Figure 4.1).
Inferior vena cava blood enters the right atrium (RA) and due to the position of the Eustachian valve, Chiari network, and FO enters the LA during 80 percent of the cardiac cycle. During the other 20 percent (atrial systole), IVC blood crosses the tricuspid valve and enters the right ventricle (RV). The overwhelming majority of superior vena cava (SVC) crosses the tricuspid valve and enters the RV as well. Blood from the RV is ejected into the pulmonary artery (PA). Approximately 10–15 percent of blood from the PA passes through the lungs to reach the LA and the rest is shunted to the distal aorta via the ductus arteriosus (DA). As a result, two-thirds of total fetal cardiac output is provided by the RV with the remaining one-third provided via the LV.
The dynamics of shunting at the level of the ductus venosus, FO, and DA result in preferential delivery of the most highly oxygenated blood to the coronary and cerebral circulations. Obviously, this preferential delivery of oxygenated blood may be compromised in utero by cardiac lesions that prevent or reduce LV output. At birth, a series circulation is established in which each ventricle pumps into a specific vascular bed (RV to pulmonary artery; LV to aorta). The removal of the placenta and the initiation of alveolar ventilation at birth have the immediate effect of establishing this series circulation. To maintain the adult series circulation, the fetal channels must be closed (Table 4.1). Complex neurochemical and hormonal influences affect the closing of these fetal shunts. Acidosis, sepsis, hypothermia, hypoxia, and hypercarbia may cause reopening of the shunts and persistence of the fetal circulation (PFC).
Table 4.1 Fetal structures and their corresponding structure in adults
Fetal structure | Adult structure |
---|---|
Foramen ovale | Fossa ovalis |
Umbilical vein | Ligamentum teres |
Ductus venosus | Ligamentum venosum |
Umbilical arteries | Medial umbilical ligaments, superior vesicular artery |
Ductus arteriosus | Ligamentum arteriosum |
Closure of the Ductus Arteriosus
In the fetus, patency of the ductus arteriosus is maintained by high levels of prostaglandin (PGI2 and PGE1). There are two stages of ductal closure in the newborn: functional closure and permanent anatomic closure. Functional closure occurs by contraction of the smooth muscle of the ductal wall, usually within the first day of life. An increase in PO2 and a decrease in prostaglandins contribute to functional closure. Oxygen is a dose-dependent ductal constrictor that acts by increasing the rate of oxidative phosphorylation within smooth muscle cells. In addition, the response to oxygen may be age-related; full-term neonates have a more dramatic response to oxygen than do immature newborns. Norepinephrine and epinephrine, by changing pulmonary and systemic vascular resistances, may secondarily contribute to ductal closure. Acetylcholine has a direct constrictor effect on ductal tissue. Permanent anatomic closure of the duct is usually accomplished by 2–3 weeks of life in the normal full-term neonate. The lumen is sealed by fibrous connective tissue, leaving the vestigial structure, known as the ligamentum arteriosum.
Closure of the Foramen Ovale
In utero, the RA pressure is higher than the LA pressure. Inferior vena cava blood flows in such a manner as to keep the FO open. Cessation of umbilical vein flow causes a significant decrease in venous return to the right heart, causing a decrease in RA pressure. In addition, ventilation causes a marked increase in pulmonary arterial and venous blood flow, resulting in an increase in LA pressure. This elevation of LA relative to RA pressure causes the flap-like valve of the FO to functionally close. In instances in which RA pressure remains elevated, right-to-left shunting may persist. Functional closure usually progresses to anatomic closure. However, probe patency of the FO may persist in 30 percent of normal adults and 50 percent of children younger than five years of age.
Closure of the Ductus Venosus
The umbilical vessels constrict strongly after mechanical stimulation and high oxygen tension facilitate this process. The resultant decrease in umbilical venous blood flow causes passive closure of the ductus venosus. The ductus venosus does not appear to be as sensitive as the ductus arteriosus to PaO2, PaCO2, or pH. The ductus venosus is functionally closed by one week of life and anatomically closed by three months. The remaining structure is the ligamentum venosum.
In addition to the establishment of the adult series circulation, dramatic alterations in pulmonary circulation, cardiac output and distribution, myocardial performance, and myocardial cell growth and hypertrophy continue to occur during the first weeks, months, and even years of life.
Pulmonary Vascular Changes
The fetus has low pulmonary blood flow secondary to a high pulmonary vascular resistance. The minimal blood flow that reaches the pulmonary bed has a very low PaO2, which may cause hypoxic pulmonary vasoconstriction and contributes to the elevated pulmonary resistance seen in the fetus. Morphologic examinations of the small arteries of the fetal and newborn lung show a thick medial smooth muscle layer. The fetal pulmonary vasculature is reactive to a number of stimulants. Vasoconstriction is induced by decreases in PaO2, pH, and leukotrienes. Acetylcholine, histamine, bradykinin, PGE1, PGE2, PGI2 (prostacyclin), and prostaglandin D2 and beta-adrenergic catecholamines are patent vasodilators of fetal pulmonary vessels.
At birth, alveolar ventilation commences. This reduces the mechanical compression of small pulmonary vessels and increases PaO2. The result is a dramatic reduction in pulmonary vascular resistance (PVR). During the following weeks and months, remodeling of the pulmonary vessels occurs; the most notable change is a thinning of the medial smooth muscle layer. By six months of life, this process results in reduction of PVR to near normal adult levels. The normal process of postnatal pulmonary maturation may be altered significantly by pathologic conditions, such as those associated with congenital heart disease.
Myocardial Performance in the Neonate
In utero, the right ventricle (RV) has a cardiac output of approximately 330 ml kg–1 min–1 compared with the left ventricular (LV) output of 170 ml kg–1 min–1. Table 4.2 summarizes the percentage of combined CO at different gestational ages [2]. At birth, both RV and LV eject an output of approximately 350 ml kg–1 min–1. This requires minimal stroke volume increase for the RV but a considerable increase in stroke volume for the LV. The high-output state of the newborn effectively limits further increases in cardiac output. This high-output state decreases to about 150 ml kg–1 min–1 by 8–10 weeks of life.
Table 4.2 Percentage of combined cardiac output at different gestational age
20 weeks | 30 weeks | 38 weeks | |
---|---|---|---|
Combined CO | 210 ml min–1 | 960 ml min–1 | 1900 ml min–1 |
Left ventricle | 47 percent | 43 percent | 40 percent |
Right ventricle | 53 percent | 57 percent | 60 percent |
Data extrapolated from [2].
Hemodynamic Changes at Birth
Myocardial morphology and performance is notably different in the neonate. These differences are listed in Table 4.3 and summarized as follows.
Table 4.3 Neonatal myocardial performance compared to the adult
Afterload mismatch |
Limited preload reserve |
Reduced contractile capacity |
Reduced ventricular compliance |
Increased intraventricular dependence |
Incomplete autonomic sympathetic innervation and dominance of parasympathetic |
Immature myocardial metabolism |
Afterload Mismatch. The neonatal heart is more susceptible to afterload mismatch, and therefore stroke volume is poorly maintained in the face of increasing outflow resistance.
Limited Preload Reserve. The neonatal heart has limited preload reserve. Augmentation of stroke volume via the Frank–Starling mechanism is limited as compared with an adult.
Reduced Contractile Capacity. Neonatal cardiac cells contain more water and fewer contractile elements than mature myocardium. In addition, there are fewer mitochondria and sarcoplasmic reticulum (SR) and poorly formed T-tubule that make the myocardium more dependent on extracellular calcium. Development of the SR, T-tubular system, and calcium-handling proteins appear to be rapid and it has been suggested that they are relatively mature by three weeks in the neonatal heart [3].
Reduced Ventricular Compliance. The compliance of the neonatal myocardium is reduced because a deficiency of elastic elements parallels the deficiency of contractile elements.
Increased Intraventricular Dependence. Changes in ventricular pressure are transmitted to the opposite ventricle via the ventricular septum more readily in the immature myocardium. Left ventricular diastolic filling is disproportionately impaired in the neonate by high RV end-diastolic pressure. This is due to a leftward shift of the intraventricular septum and a reduction in LV distensibility. Right ventricular diastolic filling is impaired to an equal extent by high LV end-diastolic pressure in neonates. This enhanced ventricular interaction is caused by reduced ventricular compliance and because, at birth, the LV and RV are of equal mass. The increased volume and pressure load experienced by the LV after birth produces relative LV hypertrophy. The normal adult LV to RV mass ratio of 2:1 is not seen until several months after birth.
Incomplete Autonomic Innervation. Sympathetic innervation, which is responsible for increasing heart rate and contractility, is incompletely developed at birth. As a result, local myocardial release of norepinephrine contributes less to increases in contractility than do increases in circulating catecholamine levels. For this reason, inotropic agents such as dopamine, the effects of which are partially mediated through release of norepinephrine from myocardial nerve endings, may have to be used in higher doses to be effective in younger patients. On the other hand, the parasympathetic system, which reflexly slows the heart, is fully functional at birth.
Immature Myocardial Metabolism. The neonatal myocardium is more dependent on anerobic metabolism than the adult heart using carbohydrates and lactate as primary energy sources. This may have a somewhat protective effect, making the neonatal myocardium more tolerant to the effects of hypoxia.
Defining Hypotension
Defining hypotension in preterm and term neonates is problematic. As a consequence, there are multiple definitions of hypotension, incorporating both mean and systolic blood pressure, defined in the pediatric anesthesia and neonatology literature. The Pediatric Advanced Life Support definition of neonatal hypotension is a systolic blood pressure (SBP) less than 60 mmHg. A survey of members of the Society of Pediatric Anesthesia and the Association of Paediatric Anaesthetists identified an SBP of 45–50 mmHg as the threshold value for hypotension in neonates [4]. A mean arterial blood pressure (MAP) less than 20–30 percent of baseline is considered hypotension by many providers. In general, neonatologists define hypotension as blood pressure below the fifth or tenth percentile for gestational and postnatal age. A consensus statement addressing the lower limit of MAP by the Joint Working Group of the British Association of Perinatal Medicine and the Research Unit of the Royal College of Physicians recommended that MAP should not drop below the infant’s gestational age in weeks, which also happens to be very close to the tenth percentile for age [5]. An absolute lower limit for mean blood pressure of 30 mmHg in infants <30 weeks’ postmenstrual age has also been suggested because a MAP <30 mmHg is associated with lower cerebral blood flow and cerebral injury [6]. Defining normal BP ranges in very low birth weight (VLBW, <1500 g) and extremely low birth weight (ELBW, <1000 g) preemies is even more complicated by the fact that ductual closure and transition from fetal circulation may be delayed. Nonetheless, BP ranges based on weight and gestational age have been defined [7–9] (Table 4.4; Figure 4.2)
Table 4.4 Blood pressure in premature infants: birth weight 600–1750 g with (2 × SD)
Age(days) | Wt (g) | |||||||
---|---|---|---|---|---|---|---|---|
600–999 | 1000–1249 | 1250–1499 | 1500–1750 | |||||
sys | dia | sys | dia | sys | dia | sys | dia | |
1 | 39(17) | 23(10) | 44(23) | 23(14) | 48(18) | 27(12) | 47(16) | 26(16) |
3 | 45(16) | 31(12) | 48(15) | 37(10) | 59(21) | 40(14) | 51(18) | 35(10) |
7 | 50(15) | 30(12) | 57(14) | 43(17) | 68(15) | 40(11) | 66(23) | 41(24) |
14 | 50(15) | 37(12) | 53(30) | 64(21) | 36(24) | 76(35) | 42(20) | |
28 | 61(24) | 46(27) | 57(30) | 69(31) | 44(26) | 73(6) | 50(10) |
Data from Handbook of Anesthesiology, Department of Anesthesiology, Perioperative and Pain Medicine, Boston Children’s Hospital.
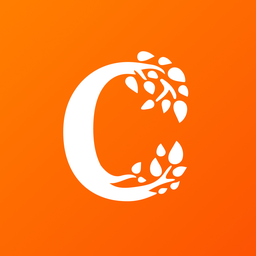
Full access? Get Clinical Tree
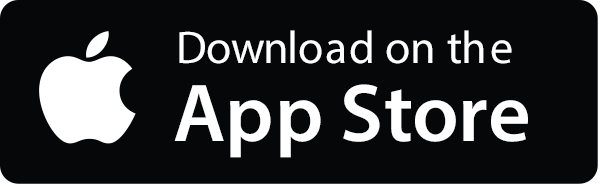
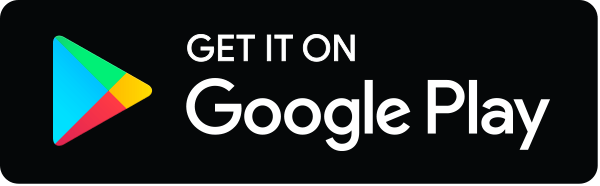
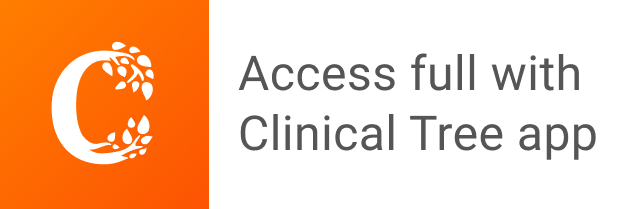