3D TEE Imaging
Annette Vegas
INTRODUCTION
The use of real-time (RT) three-dimensional (3D) TEE has become routine during the perioperative period. Ease of acquisition with the rapid online display of detailed dynamic 3D images has overcome the early limitations associated with 3D echocardiography. A basic understanding of evolving 3D technology enables the echocardiographer to master the essential skills necessary to acquire, manipulate, and interpret 3D datasets (1,2,3,4). Cardiac structures can be shown from any perspective. This single display of a moving structure is an invaluable visual aid to the echocardiographer and surgeon to better establish and communicate individual patient anatomy. Streamlined workflow and automated display options reduce the time needed in a busy operating room environment to manipulate 3D datasets for display in the virtual surgical orientation. In addition, intuitive software accessible on the ultrasound machine enables semi-automated analysis that promptly constructs static and dynamic 3D models to better quantify cardiac anatomy and function. This chapter presents key features of the 3D technology used in echocardiography and updates the current clinical applications of RT 3D TEE.
3D TECHNOLOGY
There are fundamental differences between two-dimensional (2D) and 3D echocardiographic imaging related to how the image is acquired, displayed, and interpreted. Volume scanning is used in RT 3D echocardiography as compared with the standard sector planes in 2D (5). This requires the use of special ultrasound probes to obtain raw data and integrated ultrasound machine software to process 3D datasets. Since the introduction of RT 3D TEE imaging into clinical practice in 2008, different vendors have used variations in proprietary nomenclature to describe current 3D TEE imaging (Table 21.1).
TEE Probes
The modern adult 2D TEE multiplane transducer comprises a linear phased array of 64 to 128 piezoelectric crystals arranged to cover a circular transducer face. Sequential activation of the crystals generate a 2D, sector-shaped, flat ultrasound plane that can be mechanically or electronically rotated in 1° increments through 180° to scan a conical-shaped area. 3D images can be created using a standard 2D multiplane TEE probe but this is time consuming and requires offline reconstruction.
Current commercially available 3D/4D TEE probes are similar in size and transducer frequency to the 2D TEE probes but contain thousands of crystals (Table 21.1). The Philips and GE TEE probes have a matrix
array arrangement in 50 rows by 50 columns of 2,500 piezoelectric crystals to cover the entire square transducer face. Each crystal can be independently activated (fully sampled), focused, and steered. This generates an ultrasound beam that can be steered in the azimuthal (x-y) and the elevational planes (x-z) to create a scanned 3D pyramidal volume (Fig. 21.1B). The Siemens TEE probe has proprietary technology that allows instantaneous acquisition of large 3D full volumes of the entire heart at high volume rates without the need for ECG gating. In addition, all of these 3D/4D TEE probes can simultaneously display two or more independent 2D scanning planes (biplane mode) and perform all the standard 2D functions including M-mode, Doppler modes (spectral, color, and tissue) and speckle tracking.
array arrangement in 50 rows by 50 columns of 2,500 piezoelectric crystals to cover the entire square transducer face. Each crystal can be independently activated (fully sampled), focused, and steered. This generates an ultrasound beam that can be steered in the azimuthal (x-y) and the elevational planes (x-z) to create a scanned 3D pyramidal volume (Fig. 21.1B). The Siemens TEE probe has proprietary technology that allows instantaneous acquisition of large 3D full volumes of the entire heart at high volume rates without the need for ECG gating. In addition, all of these 3D/4D TEE probes can simultaneously display two or more independent 2D scanning planes (biplane mode) and perform all the standard 2D functions including M-mode, Doppler modes (spectral, color, and tissue) and speckle tracking.
TABLE 21.1 Vendors and Equipment for 3D/4D TEE | ||||||||||||||||||||||||||||
---|---|---|---|---|---|---|---|---|---|---|---|---|---|---|---|---|---|---|---|---|---|---|---|---|---|---|---|---|
|
3D Imaging
Creation of a 3D ultrasound image of the heart involves four basic steps: (1) data acquisition, (2) data storage, (3) data processing, and (4) image display (Fig. 21.1A).
Data Acquisition
Initial 3D data acquisition obtains echocardiographic characteristics of many single points within a volume of tissue. The matrix array probe is held immobile while the transducer automatically steers the ultrasound beam to scan a pyramidal volume of tissue in three dimensions.
Data Storage
Data storage maintains data flow from the initial raw data acquisition to the next step of data processing. During the volume scanning technique, data is streamed through the on-board computer, allowing for immediate
concurrent data storage and online processing within the ultrasound machine. Offline exportation and reconstruction of the 3D dataset is no longer required.
concurrent data storage and online processing within the ultrasound machine. Offline exportation and reconstruction of the 3D dataset is no longer required.
Data Processing
Data processing consists of two integrated steps: conversion and interpolation that transforms the scanned raw data for a specific volume into a 3D dataset used to create a 3D object (Fig. 21.1C). Conversion positions and assigns x-y-z coordinates (Cartesian volume) to each of the scanned raw data points identifying their echogenic characteristics with a known position in space. Interpolation fills the gaps between all the known points in space to generate a 3D dataset comprised of voxels or volume elements. A voxel is a (vo)lume of pi(xel)s that encrypts the physical characteristics and location of the smallest cube in a dataset, which is used for 3D display. Spatial resolution of the 3D image depends on the voxel size. Larger voxels resulting in poorer resolution are generated when raw data is available for fewer points in the scanned volume and interpolation has to fill wider gaps.
The process of making a 3D dataset visible is termed 3D display which can be either as multiple 2D image planes or a 3D graphic reproduction. Graphic rendering is a computer graphics process that creates a 3D object or image. Segmentation is the first step in graphic rendering during which the object to be rendered is identified and separated from surrounding structures in the 3D dataset. This consists of differentiating the cardiac tissue from blood, pericardial fluid, and air which is facilitated by their dissimilar ability to reflect ultrasound. It is achieved by setting the threshold of echo density for blood thus excluding any point with a lower echo density from further processing. This step delineates the 3D surfaces of cardiac tissue. Following segmentation, the 3D dataset undergoes one of three increasingly complex graphic rendering techniques: wireframe, surface, or volume rendering (Fig. 21.1D-F) to display the 3D object.
3D Image Display
Wireframe rendering is the simplest and fastest way of creating a visible 3D object. A series of equidistant points on the surface of a 3D object are defined and connected with lines (wires) to form a mesh of small polygonal tiles. Smoothing algorithms can refine the narrow angles and make the object appear more real. In clinical practice, this technique is used for structures with relatively flat surfaces such as the left ventricular (LV) and the atrial cavities (Fig. 21.1D).
Surface rendering extends the wireframe technique by defining more points on the surface of a 3D object making the lines joining them invisible. It generates 3D objects with detailed solid surfaces but an empty hollow inner core (Fig. 21.1E). This makes morphologic assessment of the corresponding anatomic structure (e.g., cardiac valves) and determination of cavity volume (e.g., LV volume) feasible.
Volume rendering retains all the 3D data and displays a 3D object with full details of the surface and inner structure. This display of each voxel of the 3D dataset permits a “virtual dissection” of the 3D object (Fig. 21.1F).
Despite being composed of voxels, these 3D objects are seen on the screen as pixels of a 2D image. Perspective, light casting, and depth color coding are used to give a visual sense of depth and reality. Any rendered 3D object can be freely rotated in the display and shown from any orientation. Furthermore the 3D datasets can be displayed as a static or a moving object. A moving (dynamic) 3D object is often referred to as 4D, with time considered as the fourth dimension.
3D IMAGE ACQUISITION
All forms of ultrasound imaging are limited by the speed of sound (1,500 m/s). RT 3D TEE has a complex interdependence of temporal resolution, sector size, and spatial resolution. In 3D echocardiography, temporal resolution is described in terms of volume rate (volumes per second [VPS]) or frame rate (FR) as shown on the display. There is insufficient time for sound to traverse large tissue volumes in the 3D scanning modes which reduces FR. For instance, at a depth of 12 cm, scanning in 2D (90 scan lines) requires 14.8 ms giving a 62-Hz FR, with 3D (2,400 scan lines) it requires 396 ms resulting in a 1- to 11-Hz FR.
Currently the acquisition and display of 3D TEE images occurs in RT as either (a) single beat or (b) gated multibeat. The term “real time” refers to any 3D image that changes on the display with probe movement. A single-beat acquisition has the lowest FR and is useful with arrhythmias as it does not require an electrocardiogram (ECG). Gated images are created as a single loop by merging subvolumes acquired from consecutive heartbeats (2,4 or 6). The same portion of the ECG wave (usually the R-wave) triggers the recording of each
subvolume with rapid data reconstruction synchronous with the ECG (Fig. 21.2). It works best for a regular rhythm. Arrhythmias, electrocautery artifacts, and probe movement will affect the ability to seamlessly stitch together the subvolumes. A demarcation line, termed a stitch artifact, appears between the subvolumes distorting the anatomic structures potentially affecting analysis.
subvolume with rapid data reconstruction synchronous with the ECG (Fig. 21.2). It works best for a regular rhythm. Arrhythmias, electrocautery artifacts, and probe movement will affect the ability to seamlessly stitch together the subvolumes. A demarcation line, termed a stitch artifact, appears between the subvolumes distorting the anatomic structures potentially affecting analysis.
Imaging Modes
Single-button activation of specific 3D imaging modes for both TEE and TTE matrix array probes include (a) Live, (b) Zoom, (c) Full Volume, and (d) Color Doppler (Fig. 21.3). First-generation machine software was limited to the display of fixed-size volumes and larger adjustable 3D full volumes though not in RT. Newer technology now permits the acquisition of variable sized 3D dataset volumes (up to 120 × 120°) in all 3D modes for display in RT. Aside for the Zoom mode, the 3D dataset volume in all other modes is determined by independently adjusting the lateral or azimuthal plane (x-y) and the elevational plane (x-z) often while viewing both these planes and the 3D volume (Fig. 21.2). The volume depth is the depth of the image display. Minimizing the volume size optimizes the FR and spatial resolution. To increase temporal resolution at the same volume size, a multibeat acquisition achieves the higher volume rate.
Live mode displays adjustable-sized 3D volumes that can be rotated to easily check the general 3D image settings (Figs. 21.3A and 21.4A-C). Cardiac structures are imaged in 3D using the familiar 2D scanning planes to provide an invaluable RT guide during interventional procedures.
Zoom mode displays a magnified subsection of 3D pyramidal volume centered to a specific region of interest (e.g., the mitral valve [MV]). The acquisition involves positioning an adjustable box on two orthogonal 2D images to encompass the desired 3D volume, followed by rotation of the 3D volume to display the structure of interest (Fig. 21.3B).
Full Volume (FV) mode can be a single beat but is more often a multigated acquisition to improve temporal resolution of a large 3D volume (Fig. 21.3C). A machine preset (auto crop 50%) initially displays only half of the volume-rendered image revealing its inner details (Fig. 21.2B).
Color Doppler mode obtains a large amount of information (3D volume and 3D flow) limiting the 3D volume size and temporal resolution (low FR <20 Hz). Stitching artifacts are common as current technology requires the gated acquisition of small 3D pyramidal volumes in any 3D mode to adequately see the entire color Doppler flow in 3D. Color sectors with an appropriate Nyquist are centered on two orthogonal 2D color Doppler views to represent the 3D volume (Fig. 21.3D). The Siemens system uses proprietary technology to display large-volume 3D datasets with superimposed 3D color at high volume rates and without the need for ECG gating (Fig. 21.3E).
POSTPROCESSING
Image Optimization
Important determinants of 3D image quality and size during image acquisition are line density and transducer frequency. Line density is the number of scan lines per volume and affects the sector size and spatial resolution. A low-line density has poorer spatial resolution. Transducer frequency can be changed and as with 2D imaging can dramatically affect the image appearance. The quality of the 3D image starts with adjustment of gain and compression to optimize the 2D image. Gain, brightness (increasing whiteness) and smoothing (reducing image coarseness) are adjustable 3D settings in RT or on any stored 3D datasets (Fig. 21.4A-C).
Image Artifacts
As previously described, a stitch artifact occurs between subvolumes in an FV rendered image (Fig. 21.4D). Excessive gain in a 3D image results in noise that appears as brown speckles and may obscure structures (Fig. 21.4E). Dropout is an absence of echoes and appears black on the display (Fig. 21.4F). It may result from insufficient gain, shadowing, or ultrasound attenuation. Thin (interatrial septum [IAS]) and distant structures (aortic and pulmonic valves) remain difficult to image by 3D TEE. Most artifacts present in a 2D image will also appear in the 3D image (6).
Cropping and Orientation
A value of 3D images is the ability to crop, rotate, and display any 3D volume from any perspective (Fig. 21.5). It is now possible to present intraoperative RT 3D TEE images in the surgeon’s orientation. The reference 2D orthogonal planes can be displayed to guide orientation (Fig. 21.5C). Some vendors have reduced the
workflow so that acquisition and display can occur with single-button activation as in the case of the MV, eliminating the time needed for manipulation of the acquired volume.
workflow so that acquisition and display can occur with single-button activation as in the case of the MV, eliminating the time needed for manipulation of the acquired volume.
Cropping can be easily performed online in RT or on stored 3D images. A crop box with six standard orthogonal planes or an arbitrary cropping plane can be freely oriented in space and aligned to any anatomical structure of interest (Fig. 21.5B) to crop images.
Measurements
Current 3D technology does permit simple linear and area measurements on a 3D image. More precise measurements require exporting the 3D dataset to software to attain accurate alignment by multiplanar reconstruction (MPR) of the structure of interest. This has proven reliable and comparable to other imaging techniques such as computerized tomography (CT) and cardiac magnetic resonance imaging (MRI) for the measurement of annular dimensions, valve area, chamber volume, and components of a regurgitant color Doppler jet. Finally specific 3D quantification analysis software can create dynamic or static 3D models that improve the accuracy of existing indices and offers new indices not described by 2D TEE (7).
QUANTIFICATION ANALYSIS MODELS
Proprietary software programs are currently available for postacquisition analysis of the 3D datasets: QLAB (Philips Healthcare, Andover, MA), TomTec (TomTec, Munich, Germany), and eSie Valves(((Siemens Medical Solutions, Malvern, PA). TomTec and eSie Valves® can read 3D TTE and TEE datasets generated by different ultrasound systems while QLAB is vendor specific. All use a semi-automated method to create dynamic or static models of cardiac structures.
QLAB combines three applications (3DQ, 3DQ Advanced, and MVQ) and is available on Philips ultrasound machines and workstations for processing of 3D datasets. QLAB uses MPR to display the 3D volume in three color-coded orthogonal 2D planes: green: x/y elevation plane; red: y/z lateral plane; blue: x/z depth plane. These planes can be adjusted independently or locked together to cut the 3D dataset in an infinite number of planes, each displayed in a separate window (Fig. 21.6A).
The basic 3D quantification (3DQ, QLAB) application analyzes a LV FV dataset for quick accurate measurements of the area, length, volume, and calculation of ejection fraction (EF). The 3DQ can also be used to determine annular measurements, valve areas, and determinants of the regurgitant color Doppler jet. The advanced 3D quantification (3DQ Advanced, QLAB) application constructs a dynamic 3D endocardial cast of the LV cavity from an FV dataset (Fig. 21.6B) which can be divided into 17 LV segments (Fig. 21.6C). Mitral valve quantification (MVQ, QLAB) creates a static 3D MV model from Zoom and FV datasets for comprehensive quantification and identification of MV pathology (Fig. 21.7A).
The TomTec package includes: MV analysis (4D MV Assessment©), 3D volume quantification (4D Cardio-ViewTM), LV (4D LV analysis©), and RV (4D RV Function©) using semi-automated 3D volume reconstruction. The user interface is similar to QLAB and displays the 3D volume dataset together with three MPR. The 4D MV Assessment© provides a static and dynamic 3D MV models to analyze complex anatomy and assess MV mechanics (Fig. 21.7B). It can perform 17 segment analysis of LV function and assess LV synchronicity although its unique feature is a dynamic 3D RV model (Fig. 21.8).
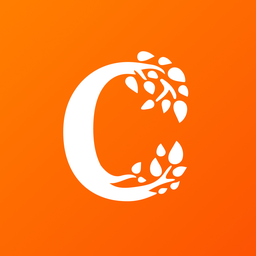
Full access? Get Clinical Tree
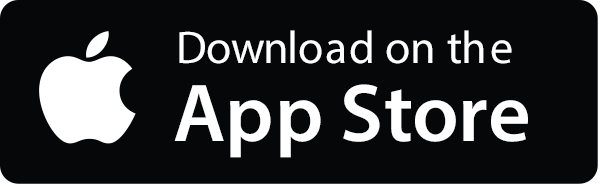
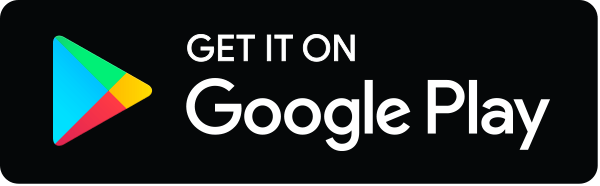