Stephen B. Horton1, Adam Skinner2, Andres Bacigalupo Landa3, Iki Adachi4, Stephen A. Stayer5, and Pablo Motta6 1 Department of Paediatrics, Faculty of Medicine, The University of Melbourne Cardiac Surgery, The Royal Children’s Hospital, Melbourne, Victoria, Australia 2 Department of Anaesthesia and Pain Medicine, The Royal Children’s Hospital, Melbourne, Victoria, Australia 3 Department of Pediatrics and Anesthesiology, Baylor College of Medicine, Arthur S. Keats Division of Pediatric Cardiovascular Anesthesiology, Texas Children’s Hospital,, Houston, TX, USA 4 Congenital Heart Surgery, Texas Children’s Hospital, Clayton Endowed Chair in Cardiac Transplant and Mechanical Support, Mechanical Circulatory Support, Texas Children’s Hospital, Department of Surgery and Pediatrics, Baylor College of Medicine,, Houston, TX, USA 5 Department of Anesthesiology and Pediatrics, Texas Children’s Hospital, Baylor College of Medicine, Houston, TX, USA 6 Staff Cardiovascular Anesthesiologist Service Lead, Adult Congenital Cardiac Anesthesiology, Arthur S. Keats Division of Pediatric Cardiovascular Anesthesiology, Texas Children’s Hospital, Associate Professor of Anesthesiology, Department of Anesthesiology, Baylor College of Medicine,, Houston, TX, USA The successful use of extracorporeal membrane oxygenation (ECMO) in children began in the late 1970s when Bartlett et al. applied this technology to term newborn infants with respiratory failure [1]. In 1973, Soeter et al. described the ECMO use for cardiorespiratory failure after tetralogy of Fallot repair [2]. Later in 1987, Kanter et al. reported the first series of 13 patients with postoperative cardiac failure treated with ECMO [3]. The utilization of ECMO for cardiac support has increased steadily since the 1980s. Improved perfusion and anticoagulation techniques make the support safer so that it may be initiated earlier and not as a last resort. Data from the Extracorporeal Life Support Organization (ELSO) reveal an increase in ECMO support of 24% from 2009 to 2015, with overall survival of 62% [4]. Neonatal cardiac, neonatal, and pediatric extracorporeal life support (ECLS) to support cardiopulmonary resuscitation (CPR) have lower survival rates. The first successful use of a left ventricular assist device (LVAD) as a bridge to heart transplant in an adult was reported by Cooley et al. in 1969 [5]. The first description of ventricular assist device (VAD) use in children was 20 years later, and the authors concluded that, as adults, VAD bridge to transplantation in children is possible [6]. In the 1990s, LVAD use in children was sporadic. However, VAD implantation in children has grown exponentially [7, 8]. Mechanical support can be considered for children with potentially reversible cardiac failure, respiratory failure, pulmonary hypertension (PHTN), and cardiorespiratory arrest. When used for cardiac failure, the primary goal is to rest the myocardium to allow recovery of function (bridge to recovery, BTR). Sometimes is difficult to estimate myocardial recovery, especially amid organ failure. In this situation, mechanical support can stabilize the patient before making a long‐term decision (bridge to decision, BTD). In the absence of recovery of the myocardium, the patient may be transitioned to long‐term mechanical support while awaiting a heart transplant (bridge to transplant, BTT) [9]. Adult patients can have a permanent mechanical device to support the circulation for the remainder of their lives (destination therapy, DT) if they are not heart transplant candidates (e.g. 70 years, malignancy, or severe comorbidities) [10]. Recently, several reports of DT in the pediatric population have been published [11]. The goal is not only to improve survival but also to improve the quality of life [12]. As with adults, pediatric patients, nontransplant candidates, such as those with muscular dystrophy, malignancy, comorbidities (e.g., severe obesity), or high allosensitization could be offered VAD as DT. The indications for mechanical circulatory support (MCS) in children are often divided into two groups: those related and those unrelated to cardiac surgery (Box 37.1). As a general principle, ECMO could potentially be used for all the indications in Box 37.1, and VAD is only considered in the absence of PHTN or respiratory dysfunction. There are pros and cons of VAD and ECMO devices, and sometimes, they are used in sequence. However, either device can be used as BTR, BTD, or BTT. The choice depends on the acuity of illness, comorbidities, the recovery potential, and anticipated duration of support [13]. In addition, if there is a choice between ECMO and VAD, a LVAD is preferable if patients are suspected of needing longer than 2 weeks of circulatory support and if the support is intended as a bridge to transplant. As ECMO has become safer, it is generally agreed that mechanical support should be initiated early before circulatory collapse to avoid prolonged periods of low cardiac output state (LCOS) and organ hypoperfusion. Patients with cardiac dysfunction after cardiopulmonary bypass (CPB) have a better prognosis when ECMO is initiated in the operating room (OR), rather than waiting for deterioration in the pediatric intensive care unit (PICU). In one study of 81 children, those placed on ECMO in the OR had a survival rate of 64%, whereas those put on ECMO in the PICU had a 29% recovery [14]. Pediatric patients who failed to wean off CPB requiring ECMO are a high‐risk population with a mortality of 55% [15]. Occasionally, neonates with severe cyanosis or cardiogenic shock require preoperative mechanical support for stabilization before cardiac surgery. There are many case reports of successful use of ECMO in this context [16]. At Royal Children’s Hospital and Texas Children’s Hospital, we have numerous successful anecdotal examples of preoperative ECMO use. At the Children’s Hospital, Boston, a retrospective review over 13 years demonstrated the use of ECMO in 26 patients with acute cardiorespiratory deterioration and unrepaired congenital heart disease (CHD) as a bridge to palliative or definitive surgery. Sixteen out of 26 patients survived to discharge. [17] Despite no studies showing superiority of this approach, it is reasonable to conclude that preoperative ECMO may be a useful rescue modality to allow cardiopulmonary and other end‐organ recovery as well as a bridge to surgery. Specifically, however, it is noted that neonates with obstructed total anomalous pulmonary venous return (TAPVR) are poor candidates for this approach as the pulmonary pressures remain unacceptably high despite mechanical support and surgery should be performed promptly [17, 18]. Despite this, however, preoperative ECMO can still be lifesaving in patients with obstructed TAPVR. Failure to wean from CPB after congenital heart surgery is the most common cardiac indication for mechanical support and it is estimated that 2–5% of all children require ECMO after congenital heart surgery [4, 19]. Extracorporeal cardiac life support may be required after CPB due to an LCOS, respiratory failure or severe hypoxemia (due to right‐to‐left shunt or occluded systemic‐to‐pulmonary artery shunts). The etiology of an LCOS after CPB may be anatomical (and must be excluded by echocardiography or sometimes catheterization), ventricular dysfunction (often due to long CPB and cross‐clamp times), arrhythmias, or PHTN [20]. Mechanical support could be required immediately on attempted separation from CPB or may be required later and used as rescue in intensive care. If mechanical support is initiated in the OR, the cannulas are often placed in the right atrium and aorta via the sternotomy. Criteria for considering ECMO or VAD in patients with LCOS include a progressive increase in inotrope or vasopressor dosage with evidence of poor end‐organ perfusion, such as reduced mixed venous oxygenation <40%, reduced cerebral saturation (>20% below baseline), oliguria, or metabolic acidosis with increasing lactate. Again, initiation of early mechanical support in these situations shows better outcomes [9]. Patients with single‐ventricle physiology or cyanotic heart disease are more likely to require mechanical support after CPB. According to the ELSO registry 2015, the most frequent CHD requiring ECMO in neonates is hypoplastic left heart syndrome (HLHS), with 33% survival of stage 1 palliation surgery, although generally those who require ECMO for hypoxia (often an occluded shunt) have a better outcome than those who require support for low cardiac output [21]. A recent systematic review and meta‐analysis of ECMO after CHD repair showed the most common reason for ECMO was left ventricular (LV) dysfunction with LCOS. About 60.3% were able to wean from ECMO and the overall in hospital mortality was 56.8%. Single‐ventricle physiology and renal failure were the only independent risk factors for in‐hospital mortality [22]. Resuscitation of children with ECMO during CPR for cardiac arrest was first described in 1992. del Nido et al. reported on the use of veno‐arterial ECMO (VA‐ECMO) for 11 children with a witnessed sudden cardiac arrest [23]. Ten out of 11 patients were centrally cannulated, and seven patients survived to hospital discharge. Using extracorporeal support for management of cardiac arrest is termed “extra‐corporeal cardiopulmonary resuscitation” (ECPR). Rapid VA‐ECMO for patients suffering in‐hospital cardiac arrest (IHCA) has been used increasingly in pediatrics since then [18, 24, 25]. The American Heart Association (AHA) 2010 resuscitation guidelines state, “There is increasing evidence that extracorporeal cardiac life support (ECLS) can act as a bridge to maintain oxygenation and circulation in selected infants and children with cardiac arrest if they are transplant candidates or have a self‐limited or treatable illness” [24]. When compared with CPR alone, it appears that ECPR improves survival by 12–23% in children [26]. This is consistent with a multivariate analysis of an observational registry study which showed higher rates of survival to hospital discharge for those managed with ECPR [27]. From analysis of the same registry, ECPR was associated with better neurological outcomes in patients with IHCA of any etiology [28]. After longer‐term follow‐up, overall, about one‐third of pediatric patients have good neurobehavioral outcomes 1 year after ECPR for IHCA [29]. The AHA pediatric resuscitation guidelines of 2020 recommend “ECPR may be considered for pediatric patients with cardiac diagnoses with IHCA in settings with existing ECMO protocols, expertise and equipment” [25, 27, 28]. ECPR is typically only effective when arrest occurs in a highly monitored environment (such as the intensive care); the AHA cautions that there is insufficient evidence for or against for the use of ECPR in out of hospital cardiac arrest or IHCA in patients with noncardiac disease [25]. The AHA (2010) states that there is no good evidence for an upper limit as to the length of CPR after which ECPR should be considered futile; it is known that long‐term survival is possible after >50 minutes of CPR [30]. A recent review of the ELSO registry for term and premature neonates receiving ECPR from 1998 to 2010 revealed 39% survived to discharge, however, gestational age, corrected gestational age, and birth weight correlated inversely with stroke and death. Of those born before 34 weeks, the survival was 21%. As expected, if dysrhythmia was the primary diagnosis, the odds of death were significantly lower than those with single‐ventricle physiology. Overall, the survival rate was like that of older children receiving ECPR [31]. The April 2021 ELSO report also revealed an overall 42% survival to discharge or transfer in neonatal and pediatric patients receiving ECPR [21]. Chapter 25 presents more information about cardiac arrest and resuscitation in CHD. When considering mechanical support for respiratory failure, neonatal patients are typically differentiated from (older) pediatric patients due to different etiologies and pathophysiology. With neonates, the conditions requiring ECMO are either acquired at birth or congenital in origin, such as meconium aspiration, pulmonary hypoplasia (possibly with congenital diaphragmatic hernia (CDH)), pneumonia/septicemia, and persistent PHTN of the newborn [32]. Of these neonatal causes, CDH and meconium aspiration syndrome (MAS) are the two most common reasons for the use of ECMO. The specific criteria for ECMO in neonates with respiratory disease vary depending on etiology and on location of providers [33, 34]. However, the general principle is to refer for ECMO due to failure to improve on optimal medical management (lung protective strategies, pulmonary vasodilators, surfactant, and high‐frequency ventilation) and the desire to avoid ventilator‐associated lung injury. The optimal timing, however, may be difficult, since ECMO is no longer seen as a last‐ditch attempt, so that, intervention is aimed at reducing morbidity as well as mortality [35]. Outcome is dependent on multiple factors, including etiology and underlying associated conditions; the 2016 ELSO registry suggests that neonates with CDH requiring ECMO have a 51% survival whereas those with MAS requiring ECMO have an overall 94% survival [4, 35]. The use of ECMO in the neonatal population decreased in the 1990s due to advances in ventilation strategies (including high‐frequency oscillation) and medical management, such as nitric oxide (NO) [36]. However, the rate of ECMO use for persistent PHTN and CDH has remained steady. Currently, in total, approximately, 800 neonates require ECMO support annually [4]. Older pediatric patients require ECMO because of acquired conditions, such as pneumonia (bacterial, viral, or fungal), aspiration, acute respiratory distress syndrome (ARDS), or acute respiratory failure from other causes [4]. Of these, infection is the leading cause of respiratory failure requiring ECMO [37]. Today, many pediatric patients requiring ECMO have significant comorbidity, such as renal, lung, and heart diseases. In an ECMO registry review, 19% of these patients had comorbidities in 1993, compared with 47% in 2007 [38]. Because of a lack of randomized controlled trials, the use of ECMO as a treatment modality has been slowly accepted, and recent evidence demonstrates accelerated interest from new advances in equipment/technique [39]. For example, veno‐venous cannulation has reduced the need for carotid puncture and therefore reduced the risk of neurological complications [38]. Veno‐venous ECMO (VV‐ECMO) has had a higher survival to discharge compared with VA‐ECMO [37]. During the severe acute respiratory syndrome coronavirus 2 (SARS‐CoV‐2) pandemic, a minority of pediatric patients with multisystem inflammatory syndrome in children (MIS‐C) required ECMO support. A systematic review of reported cases fulfilling the WHO criteria of MIS‐C showed that the need for ECMO was 4% in 783 patients [40]. The ELSO supports the early use of VV‐ECMO in ARDS due to SARS‐CoV‐2 in patients that other measures have failed, especially prone positioning [41]. Technically, ECMO could be used as a bridge to lung transplantation; however, long waiting times on ECMO are associated with morbidity and mortality from bleeding at the cannulation or surgical entry sites, hemolysis and coagulopathy, sepsis, and multiorgan failure. It is known that the survival of pediatric patients having ECMO as a bridge to heart transplant is significantly better than for lung or heart–lung transplant [42]. ECMO is therefore not favored for long‐term use as a bridge to lung transplant. New machines, such as the pumpless lung assist devices (described later) may alter the risk–benefit assessment of mechanical support in these patients. After lung transplant, patients who develop primary graft dysfunction may require extracorporeal support. Both VV‐ECMO and VA‐ECMO can be used, allowing the lungs to recover from the acute injury with an overall rate of survival to discharge of 42% [43, 44]. The updated definition of sepsis in adults is “life threatening organ dysfunction caused by a dysregulated host response to infection” and the definition of septic shock is “a subset of sepsis in which underlying circulatory and cellular/metabolic abnormalities are profound enough to substantially increase mortality” [45]. The definition of sepsis in children is currently being re‐examined, but is likely to include risk factors, clinical criteria, and illness severity scores to identify those at high risk of developing sepsis‐associated organ dysfunction and mortality [46]. In the meantime, the old definition of severe sepsis is being used in children and refers to the presence of organ dysfunction, hypoperfusion, or hypotension [47]. Sepsis is one of the leading causes of mortality and morbidity worldwide and severe infections kill more than 4.5 million children every year [48]. In Australia and New Zealand from 2007 to 2013, invasive infections, sepsis, and septic shock were responsible for 765 (26·4%) of 2,893 pediatric deaths in ICUs [48]. Overall mortality for invasive infections was 3.9%, sepsis was 5.6%, and septic shock was 17%. Identification of those children at highest risk of mortality is therefore of paramount importance. Septic shock may be the primary reason requiring mechanical support, or sepsis could be a coexisting problem in a patient with respiratory or cardiac failure. This causes classification difficulties, which can pose a problem for extracting meaningful outcome data. Despite this, sepsis was historically considered a contraindication for ECMO; however, it is now accepted that ECMO is a viable therapy in neonates and children. In neonates, the survival rates are over 80%, whereas, in older children, it is lower, but it has improved lately to 75% for refractory shock and 90% for refractory pneumonia [49]. A recent systematic review of ECMO in children with sepsis was consistent with this whereby overall pooled survival was 65% with neonatal survival the highest group at 73% [50]. Gram‐positive organisms were the most common among those requiring ECMO, although many underlying bacterial, viral, and fungal organisms can be implicated [51]. More recently, a sepsis mortality prediction model has been used to assess the effectiveness of VA‐ECMO in pediatric septic shock; in patients with a predicted mortality higher than 47.1%, the measured mortality was reduced by 16.1%, whereas in patients with a lower predicted mortality had a higher measured mortality by 16.8% [51, 52]. Some questions remain unanswered from this study, for example, the mortality prediction tool did not consider the different hemodynamic profiles of sepsis in children [53]. In addition, the mode of ECMO was not consistent; 71% provided central ECMO with higher flows compared with 29% with peripheral ECMO. There is some evidence that there is survival benefit from using ECMO with higher than 150 mL/kg/min flows [54]. The American College of Critical Care Medicine has issued a consensus statement on the management of children with septic shock, suggesting that ECMO be considered when a child is deteriorating from hypotension, rising lactate, or progressive multiorgan dysfunction despite high doses of vasoactive/inotrope medications, aggressive fluids, and appropriate medical management [50]. During this assessment, the rate of progression of shock with associated physiological decline is more important than the absolute amount of inotropic support [47]. In children without CHD, viral myocarditis is the commonest cause of acute heart failure. ECMO is the initial modality of choice for MCS, because of ease of application, biventricular support, and relatively few complications [55]. Once on mechanical support, ECMO can be used as a bridge to VAD, transplant, or recovery. ECMO in this population was first reported in 1999 with an 80% survival [55]. In the 2016 ELSO registry report, the survival rate for myocarditis requiring ECMO was higher than CHD in neonates and children [4]. Pediatric patients with myocarditis have the highest survival rate of 76%. ECMO is also used in children with dilated or restrictive cardiomyopathy to BTT. Although VA‐ECMO has traditionally been the standard management strategy for myocardiopathy, several reports have shown better survival with VAD [56]. In a prospective, multicenter, single‐group cohort study, children who underwent implantation of the Excor® Pediatric VAD as a BTT were compared with a historical control group of children who received circulatory support with ECMO [57]. The patients supported with VAD had longer survival rates than those supported on ECMO but with a high incidence of complications (e.g. bleeding, infection, and stroke). Of note, children who are on ECMO at the time of listing for cardiac transplant have a worse outcome (50% 12‐month survival) compared with those on a VAD at listing (76%) [58]. ECMO is also used for patients with early and late ventricular dysfunction after cardiac transplant. In a retrospective chart review of 100 pediatric orthotopic heart transplant recipients, 15 patients required 17 episodes of ECLS. Ten of these episodes were early (<1 month after transplant), and seven were late (>1 month). Of the 10 with premature graft failure, 8 were weaned from support with graft function recovery, 1 was retransplanted, and 1 died [59]. Late failure was due to acute rejection and had a 50% mortality. ECMO is usually the initial mode of support because of the speed of application and familiarity, as well as the ability to manage circulations with right ventricular (RV) dysfunction and high pulmonary vascular resistance (PVR) [42]. Recently, the temporary use of LVAD support via miniature axial flow pumps (Impella®) solo or combined with VA‐ECMO has been successfully used after cardiac allograft failure due to acute rejection [60]. Short‐term mechanical support for malignant arrhythmias is a temporizing measure while medical management is optimized, or ablation performed. Etiology includes postcardiotomy arrhythmia, myocarditis, or coronary ischemia. Most patients had supraventricular tachycardia (69%) that needed mechanical support to stabilize. Thirty‐three percent underwent transcatheter ablation while on ECMO, while the rest received medical treatment. The long‐term survival was 59% [61]. Improvement in skills and equipment has led to a significant number of younger, sicker patients presenting for complex interventional procedures in the catheterization laboratory. ECMO has been used as rescue therapy after a critical event (e.g. failure to wean from bypass, cardiac arrest, severe cyanosis) or prophylactically to manage an LCOS. A single‐center 15‐year retrospective review found the most common indications for cardiac catheterization while on ECMO were purely diagnostic, atrial septostomies, and stenting of vessels or shunts [20]. Patients at greatest risk include those <1 month of age with poor cardiovascular performance. Four high‐risk hemodynamic variables include: high end‐diastolic pressure, low systemic arterial saturations, low mixed venous saturations, and high pulmonary artery pressures [62]. In one reported series, 57% of children receiving ECPR in the catheterization survived to discharge [63]. Three groups of patients with PHTN candidates for ECMO support. The first group is perioperative patients with reversible PHTN, for example, neonates with total anomalous pulmonary venous drainage that PVR will improve with time after surgical correction. The second group is the severe PHTN patients at high risk of an acute pulmonary hypertensive crisis from intervention or intercurrent illness. Before the PHTN crisis, the suitability of rescue ECMO is discussed. Even centers with an expert mechanical support team report low survival with ECPR in these patients [64]. The third group includes patients with severe medically refractory PHTN who require VA‐ECMO as a bridge to heart–lung or lung transplant; however, the likelihood of survival with this approach is quoted as “minimal” and should be made on a case‐by‐case basis. A recent retrospective analysis of pediatric patients on ECMO with PHTN allowed the development of mortality models. The pre ECMO poor prognostic clinical features are (age <6 months, age >5 years, absence of pneumonia, and pH <7.12). The ECMO‐related poor prognostic markers are ECPR, neurological complications, pulmonary hemorrhage, renal replacement therapy, and metabolic acidosis [65]. Novalung® (Novalung GmbH, Hechingen, Germany) has recently developed a paracorporeal pumpless interventional lung assist device that uses a low‐resistance hollow‐fiber oxygenator. This device has been applied to patients with cardiogenic shock from PHTN, with cannulas placed via sternotomy in the pulmonary artery and left atrium. High pulmonary pressures drive the blood through the oxygenator, without the need for a mechanical pump, removing CO2 and improving oxygenation. Pulmonary pressures are reduced, which offloads the failing right ventricle, potentially allowing RV recovery [65]. Most of the research with this device has been in the adult population, but there is a need for application in pediatrics. ECMO can support both adults and children who have severe toxin exposure [66]. A recent literature review identified 46 reports from 1996 to 2012 where ECMO rescued adult and pediatric patients with ARDS or circulatory shock from poisoning. The most common intoxicants cited were beta‐blockers and calcium channel blockers. However, other drugs mentioned were ibuprofen, tricyclic antidepressants, colchicine, and chloroquine. A recent retrospective review of the ELSO registry identified 28 children who required ECMO due to toxin exposure. The in‐hospital mortality was 32%. The following features were associated with higher mortality: preECMO use of NO, lower pH, elevated pCO2 or inotrope requirement while on ECMO [67]. There is a worldwide shortage of organs, and significantly fewer cadaveric donors exist for any given child when compared to adults [68]. ECMO provides the potential to improve organ perfusion and increase the availability of viable organs suitable for transplant. Small case series in adults and adolescents have been reported [69]. Cultural, religious, and legal barriers differ depending on the country. ECMO could represent a violation of donor autonomy [70]. However, ECMO has been supported in this setting by ethicists and theologians [71]. Mechanical support improves viable organ retrieval in both brain‐dead donors and donation after cardiac death (DCD) [69, 72]. Brain‐dead donors supported with ECMO after suffering cardiac arrest or severe cardiovascular or respiratory dysfunction before organ retrieval increase the pool of donors. The timing of implementing ECLS for DCD, before or after the declaration of circulatory death, depends on the institution. The aim is to reduce warm ischemia time and improve the chance of graft survival. Firm conclusions regarding organ outcomes using this technique are challenging to draw in this population at this stage. It is difficult to provide absolute numerical “cut‐offs” as to who should categorically not be offered mechanical support. Progressively over the years, the list of absolute contraindications has been shrinking [73]. Contraindications are related to either futility treatment or high risk of death or severe long‐term morbidity. As medical equipment and techniques evolve, the risk–benefit balance changes. It is, therefore, wise to consider the suitability of individual patients on their own merits. For example, infants with HLHS were once regarded as inferior candidates for ECLS, but now, this is the most common congenital heart lesion requiring mechanical support of the circulation [74]. A temporizing approach is the “bridge to decision” process for patients with acute circulatory failure. The patient is supported to assess cardiogenic shock and the end or organ damage for 24–72 hours. Thus, transitioning to a long‐term MCS device in patients, either transplant candidates or deemed to recover [75]. The futility of mechanical support refers to the presence of a lethal anomaly, either cardiac or noncardiac in origin. Futility includes fatal chromosomal disorders, severe irreversible brain damage, or unrecoverable cardiac or respiratory injury. Of course, “unrecoverable” is patient‐ and organ‐specific, and the definition of futility in the context of ECMO remains very difficult [76]. Malignancy has also been considered under this “futile” category in the past, although the outcome of children with cancer is quite good; however, patients with neutropenic sepsis following bone marrow transplantation have a dismal prognosis. Despite this, a recent report described the successful use of ECMO in a few of these children [77]. Another group considered to be “futile” are those with advanced multiorgan failure. However, McLaren et al. describe 23 children at high risk of death from septic shock, of whom 22 had damage to at least three organ systems. All were cannulated centrally through the chest to obtain higher flow rates, and 17 patients (74%) survived to discharge. This high‐flow technique appears to confer more remarkable survival than with conventional ECMO [78]. Neonates pose a particular difficulty in defining contraindications. The ELSO registry shows that neonates have good survival for all diagnoses. However, certain neonatal subsets pose a specific risk when considered for ECMO; data from the ELSO registry indicate overall premature (<37 weeks) neonatal survival from ECMO is 31% compared with 41% in term infants [79]. Survival falls to 19% for neonates <33 weeks of gestational age [79]. Much of the concern with premature neonates and ECMO is because of the risk of developing or worsening intracranial hemorrhage (ICH), i.e. low birth weight, extreme prematurity, and pre‐existing ICH. Those with low gestational age (<32–34 weeks) are at the greatest risk. Most bleeds occur in the first 72 hours after birth, so in theory, the risk of ICH and hemorrhage extension may be less after the infant is 3 days old. Grade 3 ICH (blood causing enlargement of the ventricles) and grade 4 ICH (blood extending into the brain parenchyma) confer a poor long‐term prognosis [80]. ECMO increases the risk of extension of pre‐existing ICH, and most ECMO exclusion criteria include those with ICH grade 3 or 4. Although neonates with ICH < grade 3 have the potential for hemorrhagic extension, it is feasible to manage these patients without worsening their ICH, provided there is imaging and diligent monitoring of their coagulation status [81]. In the absence of good long‐term developmental follow‐up data, many would currently consider birth weight <1.6 kg as a valid contraindication to ECMO. Regression analysis suggested that 1.6 kg was the lowest threshold weight to achieve a 40% survival in noncardiac ECLS [82]. Although some have suggested ECLS to be beneficial in neonates >32 weeks of gestational age, many consider a gestational age <34 weeks to be a relative contraindication to ECMO. As data emerge, these thresholds change; preterm and neonates <2.5 kg still demonstrate mortality rates after cardiac surgery greater than larger neonates [83]. Examining ECPR in neonates, the survival was 21% for those <34 weeks of gestational age and 20% for those <2 kg. Yet, these findings do not support a strict age cut‐off for ECPR [31]. With the above caveats in mind, a list of contraindications is presented in Box 37.2. Futile treatment in the context of repeated or prolonged ECMO is a problematic issue. The probability of ECMO survival in children with respiratory failure reduces with the number of days on support. We also know that myocardial recovery is rare after 12 days of ECMO support after pediatric cardiac surgery. Despite this, prolonged ECLS has produced remarkable outcomes in children, so that, delineating “futility” is poorly defined. Therefore, repeat ECMO should not be offered unless the mechanical support was initially withdrawn prematurely or considered a new treatment modality [83]. Poor prognostic features include hyperkalemia in the context of hypothermic arrest. Hyperkalemia indicates irreversible cell damage, potassium leakage, and a poor chance of recovery [84]. At Royal Children’s Hospital (RCH), our ECMO program began by keeping on CPB overnight patients unable to be weaned. This effort was the last opportunity for the patient to either limp off CPB or die. Unfortunately, most failed to wean and died. Understanding how the ECMO service began gives us insight into where we are now and where we will be tomorrow. Due to the needed change in our service, a small team visited major US pediatric cardiac programs in 1988 and put together a peculiar system that was an adaptation of the CPB machine. The new design used a roller pump, silicone membrane oxygenator with a bladder on the venous line that on collapse would cause an alarm; however, not the pump to stop. The new ECMO system required perfusion bedside 24/7 assistance during all the mechanical support. Later, we modified the ECMO system using a Biomedicus centrifugal pump with pressure monitoring of the venous inlet and arterial outlet. Subsequent, in 1989, Dr Roger B. Mee implanted a modified version of the ECMO system without oxygenator configuring an LVAD in an infant post anomalous left coronary artery from the pulmonary artery (ALCAPA) repair with isolated LV dysfunction who was unable to wean off CPB [85]. The patient was well supported for 7 days and then successfully weaned. This infant was the first pediatric patient assisted LVAD as a BTR. The next step in improving the ECMO system was to adopt centrifugal pumps, which significantly improved their ease of management. However, the high‐resistance silicone oxygenator was still an Achilles heel of the circuit requiring high pump revolutions per minute (RPMs) to overcome the afterload. These resulted in high shear forces and cellular dysfunction, which promoted bleeding. Active development of improved centrifugal pumps occurred through the 1990s, with Deutschen Herzzentrum München developing the Jostra Rotaflow, a partially magnetic suspended centrifugal pump. This highly efficient device removed the requirement for an axle and seals, which were prone to thrombose and fail after 5 days, such as the Biomedicus Biopump® (Medtronic Corp., Minneapolis, MN, USA). In 1992, a trial comparing the Biomedicus Biopump (Medtronic Corp., Minneapolis, MN, USA) with a conventional roller pump found that the roller pump produced notably more hemolysis [86]. However, by day 5 of use, the Biomedicus acutely begins to cause cell trauma approaching the severity of the roller pump at 7 days. This trial confirmed the clinical experience at RCH of electively replacing the Biomedicus at day 5 as a precautionary measure or earlier if indicated. We adopted the Jostra Rotaflow (Maquet AG, Hirrlingen, Germany) in the late 1990s, which removed the need for elective pump changes. The pump rotor is suspended and driven by a radial permanent magnetic field that stabilizes the impeller in four of the six spatial degrees of freedom. It also allows it to be top‐spun on a single blood flushed pivot bearing with minimal load and friction. This pump has a small internal volume, and surface and passage time demonstrate excellent hydraulic efficiency. It can provide 10 L/min of pump flow rate against 400 mmHg of total head pressure, indicating high performance. In long‐term ECLS, or prolonged in vivo experiments, centrifugal pumps show superior blood handling characteristics if inlet pressures are monitored and do not become excessively negative [87]. Anecdotally, we have found that sub‐atmospheric pressures more than −20 mmHg (measured at the cannula) potentiate hemolysis. The concept of integrating a pump and oxygenator into a single device that can monitor core vitals become available for adults as the Cardiohelp system but did not have a pediatric equivalent [88]. The developed pediatric ECMO circuit alternatives were the PediVAS (Levitronix Technologies LLC, Waltham, Massachusetts, USA) and the Medos Deltastream DP3 (Medos Medizintechnik AG, Stolberg, Germany). The PediVAS is a fully suspended centrifugal pump designed as a midterm VAD that some centers in Europe had adopted into their ECMO circuits. However, the disposables were 20 times more expensive than the Rotaflow, which would have created too significant a financial burden in an extensive ECMO program. Our service elected to transition to the Medos Deltastream DP3 (Medos Medizintechnik AG, Stolberg, Germany) oxygenator systems. The entire circuit from Medos was approximately half the cost of the PediVAS pump head, with the potential benefits of being able to provide pulsatile flow with a more sophisticated pump controlling system. Medos provided several oxygenator sizes in a wound polymethylpentene (PMP) fiber configuration. The pump is a diagonal hybrid rotary type that rotates at approximately two times the rate of the Rotaflow to achieve the same flow. Thus, potentially making it more hemolytic if a thrombus occurs on the pump impeller. We undertook a study comparing the hemolysis rate across the three top‐performing centrifugal pumps Rotaflow (Maquet AG, Hirrlingen, Germany), Medos Deltastream DP3 (Medos Medizintechnik AG, Stolberg, Germany), and PediVAS (Levitronix Technologies LLC, Waltham, Massachusetts, USA) [89]. The analysis showed no difference between the DP3 and Rotaflow, but the PediVAS fared significantly better causing less hemolysis. Figure 37.1 (A) First ECMO circuit crafted with a roller pump and a silicone membrane oxygenator; (B) LVAD circuit in an ALCAPA patient using a BioMedicus (Eden Prairie, MN) centrifugal pump; (C) Jostra Rotaflow® (Maquet AG, Hirrlingen, Germany) centrifugal pump with the Quadrox D oxygenator; (D) Medos Deltastream DP3 (Medos Medizintechnik AG, Stolberg, Germany) with the NO delivery system incorporated in the ECMO circuit. Several companies released versions of their CPB hollow‐fiber oxygenator for ECMO use with the drawback that plasma leaked after several hours, necessitating urgent exchange. The Quadrox D oxygenator adoption finally found a device that could last for most of a potential ECMO run without failing [90]. This device was developed as a low inflammatory activating oxygenator for long CPB runs using a polymethyl pentene plate fiber rather than a spiral wind polypropylene fiber. The pores were logarithmically smaller such that wet out did not occur. Even volatile gases would not cross. The device was exceptionally good at removing even large air emboli; however, the most significant advantage was low resistance, which resulted in immediate improvement of managing anticoagulation, with significantly fewer blood products requirements during ECMO. However, this device was only available for adults, so that, initial use with neonates required a more extensive than necessary oxygenator running at lower than optimal flow, which was still significantly better than the others on the market. To reduce time to support, we also introduced leaving our circuits primed with Plasmalyte® for 4 weeks after ensuring no microbial growth or significant drop in membrane efficiency. Other companies have produced PMP oxygenators, for example, the Medos Hilite® (Medos Medizintechnik AG, Heilbronn, Germany) 7,000 LT (275 mL priming volume), 2,400 LT (95 mL priming volume), and 800 LT (55 mL priming volume). In addition, Medtronic has the Nautilus, while Spectrum is evolving their Quantum platform for ECMO. The aim is to inhibit activation of the inflammatory and coagulation systems from blood exposure to the foreign surface of the ECLS. To this end, the materials composing circuits either should be inert or hemocompatible. Hemocompatibility refers to those properties that allow ECLS circuits to maintain contact with flowing blood without producing a coagulopathy. Hemocompatibility is related to material’s surface, extrinsic conditions (e.g. cannulation sites), blood contact duration, local hemodynamic status (e.g. pulsatile vs. nonpulsatile), flow length, and diameter of tubes). For example, when surfaces feature peaks or valleys (average height or depth = 9 μm), the number of platelets adhering to polyvinylchloride (PVC) surfaces is increased three‐fold, compared with polished surfaces [91, 92]. Anticoagulation is challenging, with further improvements still required. Before, we had adopted using heparin‐coated technologies in the pursuit of reducing circuit thrombosis, which to our surprise, we found increased with its use. Unfortunately, we used tip‐to‐tip coating until a patient on VAD support developed a significant thrombus, which resulted in him dying from valve incompetence after several days of successful support. This prompted us to stop using any heparin surface coating and undertake a study investigating potential differences with heparin bonding, only to conclude that postprotamine made this technology prothrombotic [93]. Anticoagulation management has formed the most recent significant advances with less reliance on heparin as the sole mechanism. Since the 2000s, we have used prostacyclin (dose 5 ng/kg/min) as an adjunct and, more recently, NO (20 ppm) into the sweep gas of the oxygenator. These adjuncts have significantly reduced the number of circuit changes per 1,000 hours of ECMO but, more importantly, meant that patients are much less coagulopathic, meaning more stable support [94]. NO and prostacyclin have a synergistic effect on reducing platelet activation from exposure to the ECMO circuit. This reduction in circuit changes results from fewer coagulopathic patients on ECMO, resulting in stable patients requiring fewer interventions. The advantages of VAD circuits over ECMO are reduced priming volume from the lack of an oxygenator, shorter tubing, and minor trauma to blood cells. In addition, VADs lessen the ventricular work. VAD circuits are composed of inflow and outflow cannulas, a pump (intracorporeal or paracorporeal), a driving line power source, and a system controller (Figure 37.2) [95, 96]. The inflow cannula attaches to the atrium or the ventricle and transports blood from the left or right heart to the VAD. In left‐sided VAD, the outflow cannula connects to the ascending aorta; and in right‐sided VAD, the outflow cannula connects to the pulmonary artery. With the optimal placement of inflow cannulas, ventricular cannulas achieve superior unloading of the heart, reducing wall stress, allowing better ventricular recovery, and having a lower incidence of thrombosis. However, endocardial trabeculations can obstruct the inflow cannula in small ventricles (e.g. noncompaction cardiomyopathy). Therefore, these patients may require left atrial cannulation or LV cavity myectomy before ventricular cannulation. The length of planned support divides VADs into those for short‐term use (typically <2 weeks) and those for long‐term use (>2 weeks) (Table 37.1, Figure 37.3). They are also classified based on the mechanism that propels blood. For example, ventricular ejection occurs with a rotational device (e.g. centrifugal pumps), a pneumatic pusher plate (e.g. Berlin Heart), or via axial flow (e.g. HeartMate III®). Impella (Abiomed, Danvers, MA, USA) (Figure 37.4) is a micro axial flow device with three different pump sizes, at 2.5, 5, and 5.5 L/min [97]. The smaller pump provides partial left ventricle support in adults during high‐risk cardiac catheterizations (e.g., ablation procedures and urgent catheterizations for acute coronary syndrome). But in pediatrics, this smaller pump can also offer full left ventricle support. The Impella 5 and 5.5 L/min provide full left ventricle mechanical support for adolescents and adults. The main indication is BTR, BTD, or bridge to a permanent support device. The Impella is inserted retrograde through a femoral or axillary artery. The device inlet zone is resting in the left ventricle cavity, where blood is collected and propelled into the aorta. The deployment is performed under direct vision by fluoroscopy and echocardiography. Like many devices initially designed for adults, the Impella will have limited use in younger patients because of its size. The 2.5 L/min pump has a 12 F motor in diameter and requires a 13 Fr peel‐away introducer for delivery. Morray et al. in a magnetic resonance imaging (MRI) and echocardiographic study, define minimum size parameters that are necessary for the correct placement of the Impella 2.5 L/min catheter. The device needs a 7.5 cm LV catheter length achieved when the patient height is 122 cm, the weight of 23 kg, and body surface area (BSA) of 0.89 m2 [98]. The 5.0 L/min pump is a 21 F motor in diameter, requiring a femoral cutdown for delivery. The 5.5 L/min motor is 18 F in diameter, requiring axillary cutdown. The 5.5 L/min pump does not have a pigtail at the end of the catheter and is shorter (70 vs. 135 cm). Finally, another Impella, the Impella RP, is designed to provide support to the right ventricle. The indication for the Impella RP is to support the failing right ventricle percutaneously implanted RV assist device (RVAD). It is inserted in the femoral vein via a 23‐French sheath, and the motor has a 22 F diameter. Recently, Qureshi et al. published their experience with the Impella RP in adolescents with RV failure reporting an 83% survival to hospital discharge [99]. Figure 37.2 Illustration of the (A) ECMO circuit draining deoxygenated blood (negative pressure) from the RA to the pump (positive pressure) through the oxygenator back to the patient through the aortic cannula. Note the ultrafiltration through the venous side beyond the pump; (B) VAD circuit draining oxygenated blood (negative pressure) from the LA to the pump (positive pressure) back to the patient through the aortic cannula. Note the ultrafiltration beyond the pump. Abbreviations: RA, right atrium; Ao, aorta; LA, left atrium. Table 37.1 Ventricular assist device systems available in the United States See text for complete details. a Smaller patient reported 1.9 kg neonate. b 2.5 L/min flow needs a 17 F arterial cannula; 4 L/min flow needs a 15 F arterial cannula. c The Impella is a partial support device which enhances the patient cardiac output – the limitation in pediatrics is the vascular access needed for the size of the cannulas. d Depends on pump size and set rate. Figure 37.3 Algorithm of MCS used at Texas Children’s Hospital. The recall of the HeartWare Ventricular Assist System by the FDA leaves a gap in the patients with BSA ≥0.7 and <1.3. Abbreviation: BSA, body surface area; FDA, Food and Drug Administration; MCS, mechanical circulatory support. Figure 37.4 (A) Impella 2.5 L/min catheter showing the pig tail end (grey arrow), the blood inlet area (black arrow) and the blood outlet area (blue arrow); (B) Impella console placement signal in red aortic pressure and in white left ventricular pressure; and (C) Green trace showing the motor current in MA, the device flow is 2.9 L/min (white arrow). The Berlin Heart VAD (EXCOR) (Figure 37.5) is a pulsatile, paracorporeal device suitable for all pediatric patients, including neonates [100]. It is available in several sizes (10–60 mL). Most miniature pumps are appropriate to support neonates and infants (weight 3–8 kg), and the 25‐ and 30‐mL pumps will support children weighing up to 20–25 kg. It provides pulsatile flow delivered through a pneumatically driven thin membrane pump. In diastole, blood enters the pusher‐plate polyurethane chamber through an inlet valve, and negative pressure aid the pump filling. The blood‐filled pump is compressed from an air‐filled chamber in systole, creating pulsatile systolic flow ejected through the outlet valve to the aorta. Mechanical valves direct the flow, and there is no direct contact between the pumping mechanical parts and blood. The maximum systolic positive pressure generated is 350 mmHg, and the maximum negative driving pressure is −100 mmHg. High pressures are sometimes needed to overcome the resistance of small pediatric cannulas. The pump allows rates between 30 and 150 beats/min and the systolic time between 20 and 70% of the cycle; these parameters can all be monitored and adjusted on the external driving unit. The blood pump is transparent, allowing visual inspection of filling, emptying, and thrombus formation. The pump exchange is indicated if there is thrombus formation in the pump or cannulas to avoid systemic embolization. The blood‐contacting surfaces of the pump, including the polyurethane valves, are covered with Carmeda® bioactive heparin coating to prevent thromboembolic complications. The EXCOR has silicon cannulas with a Dacron covering that works as a biological barrier against ascending infections. Patients supported with this device often do not require mechanical ventilation; they can eat normally and ambulate, making intensive care unit (ICU) discharge possible [97, 98] The EXCOR can be used for biventricular support using two pumps (right VAD [RVAD] and LVAD) controlled by the same external driving unit. The one prospective trial from the USA comparing the EXCOR with ECMO as a bridge to transplantation showed better survival rates with the VAD than with ECMO in two different BSA cohorts (cohort 1, <0.7 m2; cohort 2, 0.7–<1.5 m2) [57]. Unfortunately, the incidence of serious adverse events was high in both groups, including major bleeding (42 and 50%, respectively), infection (63 and 50%, respectively), and stroke (29 and 29%, respectively) [57]. In addition, the need for pump exchanges due to thrombosis was frequent. The higher‐risk patients in the US pediatric experience had a smaller size, renal dysfunction, hepatic impairment, and required biventricular assist. ECMO before implantation and CHD was not associated with worse outcomes in this study population. In the German experience with 122 implantations, the overall median duration of support was 63.6 days (range 1–841). Fifty‐six (45.9%) were transplanted, 18 (14.7%) patients recovered and weaned, 43 (35.2%) patients died, and five remain on the device. In addition, pump exchange due to thrombus (35 patients) and re‐exploration due to bleeding (22 patients) were common complications [101]. Figure 37.5 (A) Picture of the Berlin Heart console showing: left pump settings (systolic pressure 180 mmHg, diastolic pressure – 30 mmHg, rate 65 bpm and percentage of systole of the cycle 40%). (B) Picture of a Berlin Heart LVAD. The black arrows show the blood entering the VAD from LV (LVAD) and the grey arrow show the blood leaving the VADs to the aorta (LVAD). Figure 37.6 (A) Photograph of the HeartWare Ventricular Assist System (total height 58 mm, diameter 49 mm) displaying the drive line (4.2 mm, grey arrow), inlet cannula (diameter 20.5, length 25 mm, white arrow) and outlet cannula (10 mm, black cannula); (B) Postoperative anterior–posterior chest x‐ray showing the implanted HeartWare Ventricular Assist System with drive line (grey arrow) and inlet cannula (white arrow), the outlet cannula is not observed because is not radiopaque, in addition an automatic implantable cardioverter defibrillator (AICD) and leads are observed; and (C) HeartWare Ventricular Assist System monitor displaying cardiac output (liter/minutes), device rpm, power (watts) and the graphs (power/time and flow/time). The HeartWare Ventricular Assist System® (HVAD) (HeartWare, Inc., Miami Lakes, FL) [102] (Figure 37.6) is a small intrapericardial centrifugal flow pump with a rotating impeller, forcing blood through the device using hydrodynamic and centrifugal forces. The displacement volume is 50 mL and can flow up to 10 L/min. The inflow cannula is integrated within the device and is inserted into the left ventricle apex by an adjustable sewing ring – the 10 mm outflow graft anastomoses to the ascending aorta [103]. An external console controls the pump, regulates power, monitors performance, and displays alarms. The pump is connected to the console by a subcutaneous driveline through the patient’s abdominal wall. For safety reasons, two of three different sources power the HVAD: rechargeable lithium batteries, alternating current (AC) power, or a 12 V DC power source. The worldwide experience with the HVAD in 205 pediatric patients (<18 years) showed a low mortality (10.7%) and successful BTT (65%). In addition, a small number of patients (3.2%) recovered function and were explanted. However, right heart support and pump exchange were mortality risk factors [104]. June 3rd, 2021, the US Food and Drug Administration (FDA) alerted the medical community that Medtronic stopped the sale and distribution HVAD system. The recall was due to the increased risk of neurological adverse events and mortality associated with the internal pump. In addition, there is a potential for the internal pump to stop and fail to restart. The HeartMate III (HM3) (Abbott Cardiac Arrhythmias and Heart Failure, Plymouth, MN) is a new centrifugal VAD characterized by a fully levitated rotor lacking mechanical bearings [105] (Figures 37.7 and 37.8). The HM3 design has a short and wide inflow that decreases shear stress and provides a reliable blood flow pattern supply (Figure 37.8B). In addition, HM3 incorporates a “pulsatility” feature wherein the rotor drops below the set speed and immediately increases above the set speed generating a pulse (~30 cycles per minute). The purpose of “pulsatility” is to allow ejection through the aortic valve reducing stasis and thrombosis. The HM3 insertion is intrapericardial, like the HeartWare® HVAD. Therefore, the modular driveline replacement in the event of malfunction does not need a complete pump exchange. The control screen consists of four boxes displaying pump flow, pump speed, pulsatility index (abbreviated on‐screen as pulse index), and pump power. The HM3 allows a wide range of working speeds accomplishing flows between 2.5 and 10.0 L/min. The pump flow is a calculated value. The pulsatility index value is inversely proportional to the support given. The higher the pulsatility index, the lesser the VAD support. The HM3 power ranges between 0.0 and 25.5 W. Five life‐threatening warnings display on the system monitor, including pump off, driveline disconnection, low flow × min, low voltage, and no external power. Low‐level speed is considered fewer than 3000 rpm. The miniaturized pocket‐size controllers and 17 hours battery pack life supports an ambulatory lifestyle. The pediatric experience with HM3 is limited. However, recently O’Connor et al. published the first pediatric HM3 series (40% of the patients < 60 kg), including a few with CHD (mostly Fontan circulation) who presented a low incidence of mortality and adverse events [107]. The smaller patient implanted in this series was 19 kg (BSA 0.78 m2). There are two limitations to its pediatrics use one is the safest lowest flow (2–2.5 L/min) and the inflow cannula (25 mm) that seats inside the LV chamber [108]. The traditional anticoagulation regime for HM3 includes Coumadin (INR target 2.0–3.0) plus antiaggregation with aspirin (100–200 mg daily). The MAGENTUM 1 trial showed that low‐intensity anticoagulation with Coumadin to a lower INR target (1.5–1.9) and aspirin was adequate [109]. The risk of thromboembolic complications after the stabilization period (6 weeks) did not increase. Figure 37.7 Diagrams of the HeartMate III Centrifugal‐Flow Pump: (A) shows a diagram of the fully magnetically levitated centrifugal‐flow pump with the control system and the external battery pack and (B) illustrates the blood entering the central axis of the rotor and is driven outward centrifugally to the outflow of the pump. Mehra et al. [106]. Reproduced with permission of Massachusetts Medical Society. Figure 37.8 (A) Clinical screen showing four parameter boxes at the top of the screen report measured values of pump flow (lpm), pump speed (RPM), pulsatility index (abbreviated on screen as pulse index), and pump power (Watt); (B) picture of the HeartMate III showing the inflow cannula (grey arrow), outflow cannula (white arrow), and the drive line (black arrow). SynCardia Total Artificial Heart. (SynCardia Systems Inc., Tucson, Ariz) (Figure 37.9). It is a pneumatically driven pulsatile biventricular device with two prosthetic polyurethane ventricles (70 mL each) [110]. Each ventricle has two mechanical valves providing unidirectional inflow and outflow from the ventricle. The valves are single‐leaflet Medtronic‐Hall (Minneapolis, MN), 27 mm for the inlet and 25 mm for the outlet. The SynCardia Total Artificial Heart (STAH) has the most significant inflow, and shortest distance of blood traveled of all available VADs. The large valves and short blood path provide very little resistance, decreasing stasis and thrombosis [111]. The prosthetic ventricles coupled with silicone cuffs to two atrial connectors and two connectors on the end of the grafts sewn to the aorta and pulmonary artery. The external console displays the pressure waveform of each cardiac cycle and has two independent controllers for emergency backup. Compressed air powers the device. Two separate wire‐reinforced conduits connected to the right and left prosthetic ventricles. The indication of the STAH are patients unable to be supported by VAD, such as those who require biventricular support or have PHTN (>4.5 Wood units). Successfully used after catastrophic intraoperative heart damage, failing Fontan, refractory arrhythmias, or irreversible cardiac rejection after transplantation have been reported. Pediatric use is limited because the device needs a distance between the sternum and the 10th anterior vertebral body of >10 cm. The recommended BSA is >1.7 m2, but it has been used successfully in patients with a BSA of 1.5–1.7 m2. The smaller 50 mL device is recommended for patients with a BSA of 1.2–1.7 m2 [112]. Morales et al. published the worldwide experience with STAH in 43 patients under 21 years old [113]. Most of the patients were older and received the 70 ml pump. Twenty‐five patients (58%) were successfully bridged to transplantation with a mean duration of support of 146 days.
CHAPTER 37
Mechanical Circulatory Support
Background, introduction, and history
Indications for mechanical support
Preoperative stabilization and support
Failure to wean from CPB or LCOS after cardiac surgery
Resuscitation of cardiac arrest
Respiratory failure and lung transplantation
Sepsis
Myocarditis, cardiomyopathy, and cardiac transplantation
Arrhythmias with hemodynamic compromise
Cardiac catheterization instability
Pulmonary hypertension
Intoxicants
Mechanical support to assist organ donation
Contraindications to mechanical support in children
Devices
Extracorporeal membrane oxygenation (ECMO)
The Pump (Figure 37.1)
The oxygenator
Thromboresistant surfaces
Ventricular assist devices
Pump/flow type
Stroke volume (mL)/pump speed (rpm)
Flow range (L/min)
BSA range (m2)
Device type
Short term (<14 days)
Rotaflow
Centrifugal/nonpulsatile
0–4,500 rpm
0–9.99
No minimuma
Rotational
Impella 2.5b
Centrifugal/nonpulsatile
0–50,000
0–2.5
>0.9–1.1c
Axial
Impella 5
Centrifugal/nonpulsatile
0–33,000
0–5
>1.1c
Axial
Impella 5.5
Centrifugal/nonpulsatile
0–33,000
0–5.5
>1.1c
Axial
Long term (> 14 days)
Pneumatic pulsatile ventricular assist devices
Berlin heart EXCOR
Pulsatile
12, 15, 25, 30, 50, 60 and 80 mL
Variabled
>0.2
Pusher plate
SynCardia total artificial heart
Pulsatile
50 and 70 mL
Up to 9.5
>1.7–2.5
Pneumatic‐driven chambers
Continuous‐flow ventricular assist devices
HeartMate III
Axial
3,000–9,000 rpm
0–10
>1.4
Axial
HeartWare®
Centrifugal/nonpulsatile
1,800–4,000
0–10
>1.4–2.6
Axial
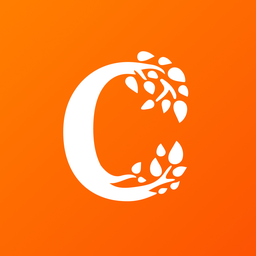
Full access? Get Clinical Tree
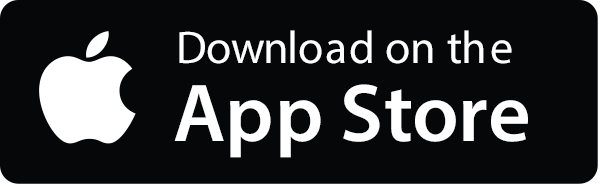
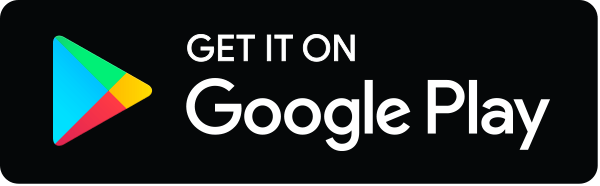