Scott G. Walker Professor of Clinical Anesthesia, Indiana University School of Medicine, Division of Congenital Cardiac Anesthesia, Riley Hospital for Children at IU Health, Indianapolis, IN, USA Left‐to‐right shunt lesions are the most common congenital heart defects, accounting for approximately 50% of all lesions. They are defined by a communication between the systemic and pulmonary circulations that allows shunting of well‐oxygenated (systemic) blood to the less‐oxygenated (pulmonary) circuit. This definition applies whether the associated structures are located on the left or right side anatomically. For instance, a child with a ventricular septal defect (VSD) and L‐transposition of the great arteries (ventricular inversion) will shunt blood from the right‐sided systemic ventricle to the subpulmonic, lower pressure left‐sided ventricle. The degree of shunting through a left‐to‐right shunt lesion may be limited by the size of the defect or the resistance to blood flow on either side. For example, shunting between high‐pressure systems like the ventricles through a large VSD is dependent on the ratio of pulmonary vascular resistance (PVR) to systemic vascular resistance (SVR). In contrast, left‐to‐right shunting through a large atrial septal defect (ASD) occurs primarily during atrial contraction and is dependent on the relative diastolic compliances of both right and left ventricles into which the atria eject. Hemoglobin concentration is another contributing factor to the amount of left‐to‐right shunting. Elevated blood viscosity, which rises with increasing hemoglobin concentration, increases both PVR and SVR. The net effect is a reduction in left‐to‐right shunting. The physiologic decline in hemoglobin concentration in the first 3 months of life is thought to have a substantial role in the normal fall of PVR after birth and may contribute to the exacerbation of symptoms related to left‐to‐right shunting. All left‐to‐right shunts produce a volume burden on the cardiovascular system, the effects of which vary according to the location of the shunt. Chamber dilation and hypertrophy, be it left, right, atrial, or ventricular, occur in structures that are involved in the pathway of the shunted volume (Figure 26.1). Shunting at the atrial level, such as through an ASD, results in an increased volume load in both atria and the right ventricle. Shunting through a VSD results in an increased volume load in both ventricles and the left atrium. Shunting at the level of the great arteries results in an increased pulmonary artery blood flow, which increases pulmonary venous return to the left atrium. The extra shunt volume dilates the left atrium and leads to increased left ventricular end‐diastolic volume and left ventricular stroke work by the Frank‐Starling mechanism. The left ventricle also gradually dilates and hypertrophies, producing increased left ventricular end‐diastolic pressure followed by increased left atrial pressure. Shunting at the level of the great arteries also produces a decrease in diastolic blood pressure from runoff of blood into the low‐pressure pulmonary circuit after the closure of the aortic valve. Low diastolic pressures decrease coronary perfusion, potentially creating ischemia from decreased myocardial oxygen delivery in the setting of increased oxygen demand from the hypertrophied ventricle. The final result is pulmonary edema from pulmonary venous congestion and left heart failure. While the right atrium and ventricle are spared the volume loading is seen in the left‐sided chambers, as PVR increases over time, there is an increased pressure burden on the right ventricle and eventual right heart failure. Figure 26.1 Schematic diagram of heart chamber dilation patterns based on level of the shunt. Black arrows represent the flow pathway of left‐to‐right shunted blood. Dashed lines represent normal chamber size. Red or blue boxes represent chamber dilation. Abbreviations: LA, left atrium; LV, left ventricle; PDA, patent ductus arteriosus. RA, right atrium; RV, right ventricle. Prolonged exposure of the pulmonary vasculature to increased flow and pressure results in a fixed increase in PVR. When PVR exceeds the SVR, the direction of the shunt can reverse and result in chronic cyanosis and erythrocytosis. Shunt reversal is more likely and occurs sooner with large defects distal to the tricuspid valve, but can be seen with any form of long‐standing left‐to‐right shunt. When the elevated PVR causing right‐to‐left shunting becomes irreversible, this is known as Eisenmenger syndrome. Figure 26.2 presents a schematic summary representation of the pathophysiology of the left‐to‐right shunting lesions and the factors that influence the degree of shunting at each level. Note that the four principal outcomes of RV failure, pulmonary edema, LV failure, and Eisenmenger physiology are possible with a long‐standing shunt at any level. For example, although shunting at the atrial level does not create a direct volume burden on the left ventricle, if it eventually produces pulmonary hypertension to a degree that right ventricle (RV) pressure exceeds left ventricle (LV) pressure, the ventricular septum will shift leftward and impair LV function. The normal compensatory mechanisms that maintain systemic cardiac output and myocardial performance in the patient with a left‐to‐right shunt include the Frank‐Starling mechanism, the sympathetic nervous system, and hypertrophy of the myocardium. Manifestations of these compensatory mechanisms include sweating and tachycardia. Infants are also often tachypneic because of decreased lung compliance associated with increased pulmonary blood flow. Tachypnea impairs feeding, and growth failure develops from both decreased caloric intake and increased caloric utilization. Although significant left‐to‐right shunts can induce heart failure, infants rarely manifest peripheral edema or jugular venous distension like adults. The most consistent sign of right‐sided failure in an infant is hepatomegaly. Anesthetic management for left‐to‐right shunt lesions should be individualized to the patient, but certain generalities do exist. Premedication with intravenous or oral drugs such as midazolam (0.05–0.1 mg/kg IV or 0.75–1.0 mg/kg PO) can be safely administered for the purpose of decreasing anxiety and providing more controlled induction of anesthesia [1]. Standard American Society of Anesthesiologists (ASA) monitors, along with the use of invasive arterial and central venous pressure monitoring and careful attention to urine output are recommended for all cases involving cardiopulmonary bypass (CBP). Trans‐esophageal echocardiography (TEE), cerebral oximetry, and cerebral blood flow monitoring are also useful monitoring adjuncts (see Chapters 14 and 15 for a more detailed discussion). Rarely, patients will present with severe, poorly controlled congestive heart failure (CHF) and be intolerant to the myocardial depressant effects of inhalational anesthetics. For this group of patients, intravenous (IV) anesthesia is preferred, with common agents including fentanyl, midazolam, and dexmedetomidine [2–5]. In most situations, however, inhalation induction with sevoflurane is a viable option when IV access is not initially available. Figure 26.2 Pathophysiology of left‐to‐right shunting lesions. The flow diagram depicts factors that affect left‐to‐right shunting at the atrial, ventricular, and great artery levels and the pathophysiology produced by these shunts. A large shunt at any level can result in RV failure, LV failure, and pulmonary edema. Increased pulmonary blood flow leads to pulmonary hypertension and eventually Eisenmenger syndrome, characterized by irreversible right‐to‐left shunting. Abbreviations: BP, blood pressure; LA, left atrium; LVEDP, left ventricular end‐diastolic pressure; LVEDV, left ventricular end‐diastolic volume; PAP, pulmonary artery pressure; PVR, pulmonary vascular resistance; R, right; L, left. See text for detailed discussion. RA, right atrium; RV, right ventricle; LV, left ventricle; RVEDP, right ventricular end‐diastolic pressure; RVEDV, right ventricular end‐diastolic volume; SBP, systemic blood pressure; SVR, systemic vascular resistance. Additional anesthetic issues include avoidance of air bubbles in IV lines to prevent paradoxical emboli. The anesthesiologist must also be cognizant of the pulmonary vasodilatory effect of oxygen and hypocarbia and manipulate ventilation in order to balance the PVR and SVR. Such measures generally include minimizing the fraction of inspired oxygen (FiO2) and avoiding hyperventilation (maintaining the pressure of carbon dioxide (PaCO2) between 40 and 50 mmHg). Isolated persistent patent ductus arteriosus (PDA) occurs in approximately 1 in 2,500 to 1 in 5,000 live births. The incidence is higher for premature births and PDA is two to three times more common in females than in males [6]. PDA is also found as part of other complex CHDs and is usually the source for pulmonary or systemic blood flow in patients with a functional single ventricle before palliative repair. The ductus arteriosus is a vascular communication between the descending aorta and pulmonary artery. Embryologically, it arises from the distal portion of one of the sixth paired aortic arches [7]. It most commonly originates from the aorta, just distal to the left subclavian artery, and attaches to the left pulmonary artery (Figure 26.3) [8]. The ductus arteriosus is an essential component in normal fetal circulation; it becomes functionally closed within 10–15 hours after birth and permanently closes by thrombosis, intimal proliferation, and fibrosis in the first 2–3 weeks. Functional closure is initiated by several mechanisms including aeration of the lungs, removal of prostaglandins produced in the placenta, increased arterial PO2, and release of vasoactive substances (bradykinins, thromboxanes, and endogenous catecholamines) [6, 7, 9, 10]. Figure 26.3 Patent ductus arteriosus. Ao, aorta; LV, left ventricle; P, pulmonary artery; RV, right ventricle. The yellow structure is the recurrent laryngeal nerve. (Source: Patrick J. Lynch, Medical Illustrator; C. Carl Jaffe, MD, Cardiologist / Wikimedia Commons / CC BY 2.5. https://en.wikipedia.org/wiki/File:Heart_patent_ductus_arteriosus.jpg.) The consequences of a PDA left untreated depend on many factors. A small PDA may be hemodynamically insignificant and unrecognized. The larger the PDA and left‐to‐right shunt, the more likely the progression to CHF, pulmonary hypertension, and if chronic and/or extreme, reversal of the shunt. In premature infants, PDA may result in increased morbidity from associated respiratory distress syndrome, necrotizing enterocolitis, and intracranial hemorrhage. As a PDA represents a shunt at the level of the great vessels, the degree of left‐to‐right shunting depends on the dimensions of the PDA, the ratio of PVR and SVR, the ratio of PA pressure to systemic blood pressure, and blood viscosity as determined by the hemoglobin level. Ductal dimensions of importance include diameter and length. Larger diameters and shorter lengths produce less resistance, with the potential to allow greater flow. In patients with large PDAs, the diastolic runoff into the pulmonary artery results in lowered aortic diastolic pressure, which may increase the risk of myocardial ischemia, especially in the presence of anemia or lowered SVR. In premature newborns, the initial management of PDA is typically pharmacological closure using cyclo‐oxygenase inhibitors such as ibuprofen, indomethacin, or acetaminophen [11, 12]. Cyclo‐oxygenase inhibitors reduce the synthesis of prostaglandins, which would otherwise act to keep the PDA. Surgical treatment is usually reserved for patients who fail medical therapy. Surgical options include posterolateral thoracotomy with ligation or division of the PDA [13] (Figure 26.4), video‐assisted thoracoscopic surgery (VATS), and robotically assisted total endoscopic closure [14]. These approaches have mortality approaching 0% and minimum morbidity; however, mortality rates in premature neonates are slightly higher. Complications of surgical treatment include bleeding, chylothorax, vocal cord paralysis (injury to the recurrent laryngeal nerve), pneumothorax, atelectasis, recurrence of patency, and inadvertent ligation of the pulmonary artery or descending aorta. Advantages of VATS compared to thoracotomy include decreased pain, decreased hospital cost (secondary to decreased hospital stay), and avoidance of post‐thoracotomy syndrome (rib fusion, chest wall deformities, scoliosis, and compromise of pulmonary function). Disadvantages of VATS include intraoperative oxygen desaturation and hypercarbia, as well as higher morbidity during the surgical learning curve [15–17]. The use of robotic assistance achieves similar outcomes but with longer surgical times because of increased complexity [14, 18]. Figure 26.4 Surgical ligation of a patent ductus arteriosus (PDA) via thoracotomy. (A) The PDA has been dissected and secured with ligatures, (B) Divided and oversewn PDA. Abbreviations: Ao, aorta; PDA‐Ao, aortic end of PDA; PDA‐PA, pulmonary artery end of PDA. (Source: Reproduced with permission from CTSNet.org.) While surgical techniques are still employed, PDA closure is now most commonly accomplished in the cardiac catheterization laboratory. Techniques include the use of a variety of devices, including coils, vascular plugs, and umbrella‐type occluders. Risks of transcatheter approaches include arrhythmias, embolization of the device, and incomplete closure [19–23]. However, these methods are considered safe, efficacious, and cost‐effective when compared to surgical closure [24, 25]. Historically, there have been size limitations for use in small infants, but newer devices may allow for transcatheter closure in patients less than 1 kg with favorable anatomy [18–23]. See Chapters 19 and 34 for further discussion of transcatheter PDA occlusion. The anesthetic management for PDA ligation depends on factors such as the patient’s clinical condition, prematurity, coexisting disease, body weight, and surgical technique. Large volume venous access (which may be a 22‐ or 24‐gauge IV in a premature infant) and forced air‐warming devices are recommended. Pulse oximetry of both upper and lower extremities will assist in detecting inadvertent ligation of the descending aorta. For patients with coexisting disease, intra‐arterial pressure monitoring provides a method of assessing arterial blood gases, electrolytes, hematocrit, and acid‐base status. Whether by cuff or arterial line, blood pressure should be monitored in both an upper and lower extremity and observed carefully before and after ductal occlusion. Proper ductal occlusion will typically be accompanied by an increase in diastolic blood pressure consistent with the elimination of pulmonary runoff. Significantly decreased or absent blood pressure in the lower extremity indicates aortic rather than ductal occlusion. A gradient between the systolic pressure in the upper and lower extremities indicates the creation of aortic coarctation. Although inhaled anesthetics can be safely used for many patients undergoing PDA ligation, neonates are prone to hemodynamic instability with exposure to inhaled anesthetics and benefit from an intravenous anesthetic technique using opioids such as fentanyl and possibly a benzodiazepine along with muscle relaxation. Neonatal PDA ligation is often performed in the newborn intensive care unit (ICU) to avoid the additional risks of transport, need for ventilator changes, and hypothermic exposure. High spinal anesthesia, caudal and thoracic epidural techniques have all been described as safe and producing faster recovery [26, 27]. Lung isolation improves surgical exposure, especially through VATS surgical techniques, but it may require ventilation with 100% inspired oxygen to maintain acceptable oxygenation. Prior to lung isolation, FiO2 should be minimized and hypocarbia avoided in order to maintain pulmonary vascular tone and to limit the degree of left‐to‐right shunting. This technique is usually unnecessary for small infants, with either gentle retraction and packing in the case of open thoracotomy or gentle CO2 insufflation with retraction for VATS, being sufficient. Infants having thoracotomy or VATS often require postoperative mechanical ventilation, especially if they are premature. Older patients or patients undergoing transcatheter closure are often extubated at the conclusion of the case [17–27]. Aortopulmonary window (APW), also known as aortopulmonary (or aorticopulmonary) fistula, fenestration, or septal defect is a rare anomaly comprising approximately 0.1–0.6% of all congenital heart defects [28–30]. A total of 50–80% of patients with APW have associated defects including PDA (72%), a right pulmonary artery from the aorta (32%), anomalous origin of a coronary artery from the pulmonary artery (23%), VSD (20%), agenesis of the ductus arteriosus (20%), and other lesions [28, 31]. Embryologically, APW is thought to originate from nonfusion or malalignment of the aortopulmonary and truncal septi, or complete absence of the aortopulmonary septum [28, 32]. The basic anatomical defect in APW consists of a communication between the aorta and the pulmonary artery. Type I APW is a proximal defect located just above the sinus of Valsalva, a few millimeters above the semilunar valve. Type II is a distal APW located in the uppermost portion of the ascending aorta. Type III is a total defect involving the majority of the ascending aorta. Type IV or intermediate defects, which are neither proximal nor distal, are also designated in this system; these defects are noted for being conducive to device closure because of adequate superior and inferior rims (Figure 26.5) [33]. Uncorrected APW results in a reported 40% mortality in the first year of life, with a substantial proportion of survivors succumbing to CHF later in childhood [32]. Figure 26.5 Classification of aortopulmonary window variants (see text for explanation). (Source: Backer [33]. Reproduced with permission of Elsevier.) The pressure gradient between the aorta and pulmonary artery will produce significant left‐to‐right shunting depending on the size of the defect and the relative resistances of the pulmonary and systemic vascular beds. A defect such as an APW is considered “restrictive” when it is small enough that there is a size‐related flow limitation across the defect indicated by a significant pressure gradient from one side to the other. Flow across a “non‐restrictive” APW is not limited by size; any pressure gradient across the defect is related only to the relative resistances of the pulmonary and systemic vascular beds. Coexisting cardiac anomalies may alter the pathophysiology. Pulmonary hypertension can develop as early as 12 days of age [34]. A variety of techniques have been described for repair of APW, including ligation and/or division with or without cardiopulmonary bypass, transaortic patch closure, complete separation, and reconstruction of both the aorta and pulmonary artery, and transcatheter closure [33, 35–37]. The repair is usually performed via median sternotomy with the use of cardiopulmonary bypass. Surgical repair of the aortic defect can be accomplished using a pulmonary artery flap with subsequent repair of the pulmonary artery with a pericardial patch [38, 39]. A Gore‐Tex® cardiovascular patch can also be used to close the defect [33] (Figure 26.6). Care must be taken to explore and repair associated anomalies of the pulmonary and coronary arteries and to repair coexisting cardiac abnormalities. Deep hypothermia and circulatory arrest may be required for the repair of large APW involving the proximal ascending aorta in neonates. Figure 26.6 Patch closure of type III aortopulmonary window (APW) total defect. Aortic cross‐clamp and cardiopulmonary bypass cannulas not shown (Source: Backer [33]. Reproduced with permission of Elsevier.) Actuarial survival after repair of APW is between 80 and 100%, with the most significant risk factor in the modern era being the presence of associated cardiac defects. Simply repaired APW lesions have a mortality approaching zero [28]. Transcatheter closure of APW is typically accomplished with an umbrella‐type occluding device [36, 37, 40–42]. Although transcatheter closure has generally been reserved for patients with restrictive defects, device closure has been reported in selected non‐restrictive defects as well [43]. The anesthetic management of APW is similar to that of truncus arteriosus. Younger patients may have considerable diastolic runoff from low PVR. Prior to cardiopulmonary bypass, efforts should focus on maintaining pulmonary vascular tone. This can be accomplished by lowering the minute ventilation and restricting the FiO2 to maintain a moderate hypercarbic respiratory acidosis and an oxygen saturation level of 80–85%. In these cases, surgical snaring of the pulmonary artery prior to bypass may also be helpful. In contrast, patients undergoing later repair are likely to present with elevated PVR, and anesthetic management should include avoiding further increases in PVR. Regardless of PVR at presentation, all patients with APW are at risk to develop perioperative pulmonary hypertension. The administration of inhaled nitric oxide, as well as other maneuvers described above, may be necessary to lower PVR. Patients exhibiting signs of pulmonary hypertension should initially be maintained under deep sedation with or without neuromuscular blockade during the immediate postoperative periods (see Chapter 33). Atrial septal defects (ASDs) make up approximately 5–10% of all CHDs with the secundum ASD comprising nearly 80% of all ASDs. Isolated ASDs are more common in females than males by a factor of 2 : 1 [9]. A probe‐patent foramen ovale is found in approximately 30% of otherwise normal adult hearts [44]. When associated with other CHDs, an ASD may be a life‐saving communication allowing mixing of blood between the pulmonary and systemic circulations. Examples include total anomalous pulmonary venous return, tricuspid atresia, and transposition of the great arteries (TGA). In such cases, an ASD may be iatrogenically created as a palliative measure, often on an urgent or emergent basis (e.g. balloon atrial septostomy). The right and left atria are normally divided by the fusion of two septa: the septum primum and the septum secundum. The septum primum develops during the fourth week of gestation and the septum secundum during the fifth week [7]. The septum primum originates posteriorly and advances across the atrial midline. The opening it leaves by incompletely advancing to the endocardial cushion is the ostium primum, which later closes. A second opening, the ostium secundum, forms in the central portion of the septum primum. The septum secundum originates anteriorly and to the right of the septum primum and advances over the ostium secundum. Although traditionally the septum secundum has been described in terms similar to those of the septum primum in its formation and composition, it is instead to a large extent composed of an infolding of the superior margin of the atrium rather than an advancing wall of tissue [45]. It eventually develops a central opening called the foramen ovale. The septum primum forms the valve of the foramen ovale on the left atrial side [7, 46]. Figure 26.7 illustrates the process of embryologic development of the atrial septum. Errors in this process form the basis for the five different types of ASDs: secundum, primum, sinus venosus, coronary sinus, and patent foramen ovale (PFO) (Figure 26.8) [48]. The secundum ASD is contained within the area bordered by the limbus of the fossa ovalis [49]. It results from abnormal reabsorption of the septum primum or defective formation or shortening of the septum secundum. Combinations of these abnormalities may contribute to large defects. The primum ASD results from abnormalities in the formation of the septum primum. It is frequently associated with atrioventricular canal (AVC) defects, especially partial atrioventricular canal (PAVCs) that include a cleft in the anterior leaflet of the left atrioventricular valve. AVC defects are due to abnormalities in the fusion of the endocardial cushions. Figure 26.7 Embryologic development of the interatrial septum. The left column is a long axis cross‐section similar to that of an echocardiographic four‐chamber view. The right column is the view from the right atrium with the right atrial free wall removed, looking at the right side of the developing atrial septum. (A) 29 days gestation. (B) 37 days gestation. (C) Birth. (Source: Adapted from Radiologykey.com.) Figure 26.8 Types of atrial septal defects. Coronary sinus ASDs are not typically visible from the right atrium, as they result from unroofing of the coronary sinus as it courses along the posterior wall of the left atrium. Also not shown is the patent foramen ovale (PFO), which is usually covered by the remnant of the septum primum. (Source: Conti et al. [179]. Reproduced with permission of Elsevier.) Sinus venosus defects result from abnormal development of the septum secundum or the sinus venosus (the primitive venous collecting chamber). The most common type is located near the superior vena cava (SVC) orifice and is associated with partial anomalous pulmonary venous return involving the right upper and middle pulmonary veins. Defects near the orifice of the inferior vena cava (IVC) also exist and may involve partial anomalous pulmonary venous return of the right lower pulmonary vein. Coronary sinus ASD, also called an unroofed coronary sinus, results from an absence in the wall between the coronary sinus and the left atrium. This allows blood from the left atrium to drain into the right atrium via the coronary sinus. Persistent left SVC is also associated with this defect [49]. PFO results from failure of fusion of the septum primum to the limbus of the septum secundum. Patency of the foramen ovale is normal during fetal life and allows right‐to‐left shunting of blood in order to bypass the lungs in fetal circulation. Following birth, PVR decreases and SVR increases. Subsequent higher pressure in the left atrium causes the septum primum to close over the foramen ovale, but it may not completely fuse to the septum secundum. Isolated ASDs are usually asymptomatic during infancy and childhood despite the increased volume load on the right ventricle. CHF usually occurs after the second or third decade of life due to chronic right ventricular volume overload. Pulmonary hypertension can occur in up to 13% of unoperated patients younger than 10 years of age; however, progression to Eisenmenger syndrome is unusual [9]. The risk of arrhythmia is increased with increasing shunt volume and atrial dilation. Patients with a Qp : Qs of 2 : 1 or less have an 11% incidence of atrial arrhythmia, compared to 38% in those with Qp : Qs of 3 : 1 or greater [50]. An ASD is sometimes discovered during a neurological work‐up for transient ischemic attacks or strokes from paradoxical emboli [49]. The amount of left‐to‐right shunting at the atrial level is dependent on two factors: the size of the defect and the relative compliance of the right and left ventricles. Shunting occurs primarily during diastole and produces a volume burden on the cardiovascular system that is proportionate to the degree of shunting. Surgical repair of an ASD is usually recommended between the ages of 3 and 5 years [51]. Spontaneous closure of small secundum type ASDs occurs in up to 87% of infants in the first year of life [9], and controversy exists regarding the closure of small ASDs that are asymptomatic. Conventional surgical treatment involves median sternotomy with the use of CPB to perform a primary repair or patch closure, with surgical mortality approaching 0% [9, 52]. Primum ASD repairs typically require patch closure as well as repair of the coexisting mitral valve cleft (Figure 26.9). Sinus venosus defects are usually repaired using a patch to close the ASD and baffle the anomalous pulmonary veins to the left atrium. The SVC may need to be translocated and anastomosed to the right atrial appendage to avoid baffle obstruction (Warden procedure). Many centers now favor partial sternotomy approaches because of the improved cosmetic result with similar morbidity and mortality to complete sternotomy [53–55]. Robotically assisted totally endoscopic ASD repair with remote access perfusion (using femoral cannulation) has been described in adults [56]. With conventional repair, postoperative dysrhythmias are reported in 23% of patients, and as many as 2% of patients may need a pacemaker following surgery [9]. Increased use of transcatheter ASD closure in the cardiac catheterization laboratory has dramatically reduced the number of operative repairs. While the several FDA‐approved devices that have been utilized differ in detail, the typical design consists of a catheter‐deployed expandable “double umbrella” type occluder, with a right and left atrial side (Figure 26.9) [57]. Nonsurgical transcatheter closure of PFO without an occluding device has also been described, using either radio frequency ablation or suture closure [58, 59]. Transcatheter ASD closure is usually performed under general anesthesia with the use of TEE to guide placement. However, intracardiac echocardiography using intravascular two‐dimensional imaging may eliminate the need for TEE and reduce the need for general anesthesia [60]. Transcatheter closure is safe, associated with decreased hospital stay, lack of a surgical scar, avoidance of CPB, and reduced anesthetic requirements. Limitations to transcatheter closure of ASD include patient size (introducer sheaths may be too large for smaller patients), type of ASD (usually limited to PFO or secundum), and the requirement for an adequate tissue rim to which the device can attach [61]. Occasionally, pre‐procedure transthoracic echocardiography does not provide complete information about septal tissue rim size or other requirements for device closure, and backup surgical repair can be arranged during the same anesthetic if the TEE examination reveals contraindications to a transcatheter procedure. Figure 26.9 Surgical repair of primum (top) and secundum (middle) atrial septal defect (ASD), and device closure of secundum ASD (bottom). A primum ASD requires closure of the mitral valve cleft (top left), followed by patch closure of the ASD (top right). A secundum ASD may be repaired by direct suture closure (middle left) or by patch closure (middle right). Secundum ASD closure device is delivered via catheter across the ASD, wherein the left atrial side of the device is opened. The device is then pulled back against the ASD, where the right atrial side is opened and the device is deployed from the catheter. (Source: Conti et al. [47]. Reproduced with permission of Elsevier.) Patients with an isolated ASD are generally asymptomatic and do not have pulmonary hypertension. Therefore, the induction of anesthesia can be safely accomplished with either inhalation or intravenous techniques. Whenever possible, patients should have an intraoperative TEE performed prior to incision, because transthoracic echocardiographic studies are sometimes unable to exclude the possibility of a partial anomalous pulmonary venous return due to difficulty in visualizing all four pulmonary veins. During surgery, TEE can be helpful to assess the de‐airing of the left heart and adequacy of the repair. Most patients have a good myocardial function and do not require inotropic support perioperatively. Maintenance of anesthesia typically consists of a combination of inhaled and intravenous agents. Adjunct regional techniques are favored by some and may facilitate early extubation [62–65]. Tracheal extubation in the operating room (OR) has been shown to decrease patient charges without compromising patient care when compared to extubation in the ICU [66, 67]. Whatever technique is chosen, the primary goals for the uncomplicated ASD patient should include preparation for early extubation either in the OR or within the first 4 hours postoperatively.
CHAPTER 26
Anesthesia for Left‐to‐Right Shunt Lesions
Introduction
Patent ductus arteriosus
Incidence, anatomy, and natural history
Pathophysiology
Surgical and transcatheter approaches and outcomes
Anesthetic considerations
Aortopulmonary window
Incidence, anatomy, and natural history
Pathophysiology
Surgical and transcatheter approaches and outcomes
Anesthetic considerations
Atrial septal defects
Incidence
Anatomy
Secundum ASD
Primum ASD
Sinus venosus ASD
Coronary sinus ASD
Patent foramen ovale
Natural history
Pathophysiology
Surgical and transcatheter approaches and outcomes
Anesthetic considerations
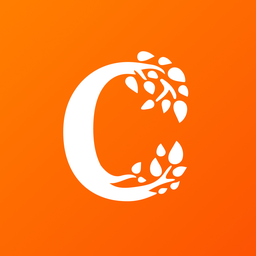
Full access? Get Clinical Tree
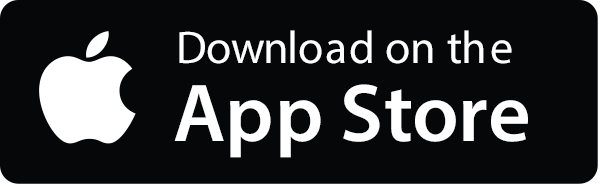
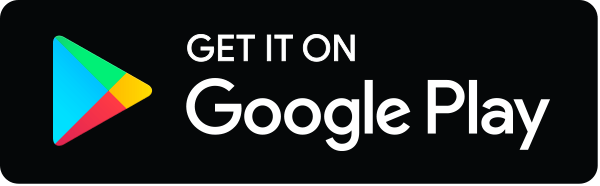