The autonomic nervous system (ANS) includes that part of the central and peripheral nervous system concerned with involuntary regulation of cardiac muscle, smooth muscle, glandular and visceral functions.
The sympathetic and parasympathetic nervous systems (SNS, PNS) affect cardiac pump function in three ways: (1) by changing the rate (chronotropism), (2) by changing the strength of contraction (inotropism), and (3) by modulating coronary blood flow.
SNS nerves are by far the most important regulators of the peripheral circulation.
The ANS can be pharmacologically subdivided by the neurotransmitter secreted at the effector cell: Acetylcholine (ACh) released by the PNS, and the catecholamines epinephrine (EPI) and norepinephrine (NE), which are the mediators of peripheral SNS activity.
An agonist is a substance that interacts with a receptor to evoke a biologic response. An antagonist is a substance that interferes with the triggering of the response at a receptor site by an agonist.
The adrenergic receptors are termed adrenergic or noradrenergic, depending on their responsiveness to epinephrine (EPI) and norepinephrine (NE).
The numbers and sensitivity of adrenergic receptors can be influenced by normal, genetic, and developmental factors.
The autonomic nervous system reflex comprises (1) sensors, (2) afferent pathways, (3) CNS integration, and (4) efferent pathways to the receptors and efferent organs.
The clinical application of ANS pharmacology is based on the knowledge of ANS anatomy, physiology, and molecular pharmacology.
Clinically, anticholinesterase drugs may be divided into two types: the reversible and nonreversible cholinesterase inhibitors.
The net physiologic effect of a sympathomimetic agent is usually defined by the relative actions on the α, β, and dopaminergic receptors.
Dexmedetomidine is a more selective α2 agonist than clonidine.
Drugs that bind selectively to α-adrenergic receptors block the action of endogenous catecholamines or moderate the effects of exogenous adrenergics.
Calcium channel blockers are not true pharmacologic antagonists of calcium. They interact with the cell membrane to control the intracellular concentration of calcium.
Multimedia
Catecholamine Chemical Structures
Renin Angiotensin
Antimuscarinics
Reversible Anticholinesterases
ANESTHESIA AND THE AUTONOMIC NERVOUS SYSTEM
Anesthesiology is the practice of autonomic medicine. Drugs that produce anesthesia may also have potent autonomic side effects. The greater part of our training and practice is spent acquiring skills in utilizing or averting the autonomic nervous system (ANS) side effects of anesthetic drugs under a variety of pathophysiologic conditions. The success of any anesthetic depends upon how well homeostasis is maintained. The anesthetic chart reflects ANS function.
The ANS includes that part of the central and peripheral nervous system concerned with involuntary regulation of cardiac muscle, smooth muscle, and glandular and visceral functions. ANS activity refers to visceral reflexes that function below the conscious level. The ANS is also responsive to changes in somatic motor and sensory activities of the body. The physiologic evidence of visceral reflexes as a result of somatic events is abundantly clear. The ANS is therefore not as distinct an entity as the term suggests. Neither somatic nor ANS activity occurs in isolation.1 The ANS organizes visceral support for somatic behavior and adjusts body states in anticipation of emotional behavior or responses to the stress of disease. In brief, it organizes fight or flight responses.
Afferent fibers from visceral structures are the first link in the reflex arcs of the ANS, and may relay visceral pain or changes in vessel stretch. Most ANS efferent fibers are accompanied by sensory fibers that are now commonly recognized as components of the ANS. However, the afferent components of the ANS cannot be as distinctively divided as can the efferent nerves. ANS visceral sensory nerves are anatomically indistinguishable from somatic sensory nerves. The clinical importance of visceral afferent fibers is closely implicated in the management of chronic pain states.
FUNCTIONAL ANATOMY
The ANS falls into two divisions by anatomy, physiology, and pharmacology. Langley divided this nervous system into two parts in 1921. He retained the term “sympathetic” (sympathetic nervous system, SNS) introduced by Willis in 1665 for the first part, and introduced the term “parasympathetic” (parasympathetic nervous system, PNS) for the second. The term ANS was adopted as a comprehensive name for both. Table 15-1 lists the complementary effects of SNS (adrenergic, sympathetic) and PNS (cholinergic, parasympathetic) activity of organ systems.
TABLE 15-1. HOMEOSTATIC BALANCE BETWEEN ADRENERGIC AND CHOLINERGIC EFFECTS
Central Autonomic Organization
Pure central ANS versus somatic centers are not known. Integration of ANS activity occurs at all levels of the cerebrospinal axis. Efferent ANS activity can be initiated locally and by centers located in the spinal cord, brain stem, and hypothalamus. The cerebral cortex is the highest level of ANS integration. Fainting at the sight of blood is an example of this higher level of somatic and ANS integration. ANS function has also been successfully modulated through conscious, intentional efforts demonstrating that somatic responses are always accompanied by visceral responses and vice versa.
The principal site of ANS organization is the hypothalamus. SNS functions are controlled by nuclei in the posterolateral hypothalamus. Stimulation of these nuclei results in a massive discharge of the sympathoadrenal system. PNS functions are governed by nuclei in the midline and some anterior nuclei of the hypothalamus. The anterior hypothalamus is involved with regulation of temperature. The supraoptic hypothalamic nuclei regulate water metabolism and are anatomically and functionally associated with the posterior lobe of the pituitary (see Interaction of Autonomic Nervous System Receptors). This hypothalamic–neurohypophyseal connection represents a central ANS mechanism that affects the kidney by means of antidiuretic hormone. Long-term blood pressure control, reactions to physical and emotional stress, sleep, and sexual reflexes are regulated through the hypothalamus.
The medulla oblongata and pons are vital centers of acute ANS organization. Together, they integrate momentary hemodynamic adjustments and maintain the sequence and automaticity of ventilation. Integration of afferent and efferent ANS impulses at this central nervous system (CNS) level is responsible for the tonic activity exhibited by the ANS. Tonicity holds visceral organs in a state of intermediate activity that can either be diminished or augmented by altering the rate of nerve firing. The nucleus tractus solitarius, located within the medulla, is the primary area for relay of afferent chemoreceptor and baroreceptor information from the glossopharyngeal and vagus nerves. Increased afferent impulses from these two nerves inhibits peripheral SNS vascular tone, producing vasodilation; it also increases vagal tone, producing bradycardia. Studies of patients with high spinal cord lesions show that a number of reflex changes are mediated at the spinal or segmental level. ANS hyperreflexia is an example of spinal cord mediation of ANS reflexes without integration of function from higher inhibitory centers.1
Peripheral Autonomic Nervous System Organization
The peripheral ANS is the efferent (motor) component of the ANS and consists of the same two complementary parts: The SNS and the PNS. Most organs receive fibers from both divisions (Fig. 15-1). In general, activities of the two systems produce opposite but complementary effects (see Table 15-1). A few tissues, such as sweat glands and spleen, are innervated by only SNS fibers. Although the anatomy of the somatic and ANS sensory pathways is identical, the motor pathways are characteristically different. The efferent somatic motor system, like somatic afferents, is composed of a single (unipolar) neuron with its cell body in the ventral gray matter of the spinal cord. Its myelinated axon extends directly to the voluntary striated muscle unit. In contrast, the efferent (motor) ANS is a two-neuron (bipolar) chain from the CNS to the effector organ. The first neuron of both the SNS and PNS originates within the CNS but does not make direct contact with the effector organ. Instead, it relays the impulse to a second station known as an ANS ganglion, which contains the cell body of the second ANS (postganglionic) neuron. Its axon contacts the effector organ. Thus, the motor pathways of both divisions of the ANS are schematically a serial two-neuron chain consisting of a preganglionic neuron and a postganglionic effector neuron (Fig. 15-2).
FIGURE 15-1. Schematic distribution of the craniosacral (parasympathetic) and thoracolumbar (sympathetic) nervous systems. Parasympathetic preganglionic fibers pass directly to the organ that is innervated. Their postganglionic cell bodies are situated near or within the innervated viscera. This limited distribution of parasympathetic postganglionic fibers is consistent with the discrete and limited effect of parasympathetic function. The postganglionic sympathetic neurons originate in either the paired sympathetic ganglia or one of the unpaired collateral plexuses. One preganglionic fiber influences many postganglionic neurons. Activation of the SNS produces a more diffuse physiologic response rather than a discrete, localized effect. GI, gastrointestinal.
FIGURE 15-2. Schematic diagram of the efferent ANS. Efferent impulses are integrated centrally and sent reflexly to the adrenergic and cholinergic receptors. Sympathetic fibers ending in the adrenal medulla are preganglionic, and acetylcholine (ACh) is the neurotransmitter. Stimulation of the chromaffin cells, acting as postganglionic neurons, releases epinephrine (EPI) and norepinephrine (NE).
Preganglionic fibers of both subdivisions are myelinated with diameters of less than 3 mm.1 Impulses are conducted at a speed of 3 to 15 m/s. The postganglionic fibers are unmyelinated and conduct impulses at slower speeds of less than 2 m/s. They are similar to unmyelinated visceral and somatic afferent C fibers (Table 15-2). Compared with the myelinated somatic nerves, the ANS conducts impulses at speeds that preclude its participation in the immediate phase of a somatic response.
TABLE 15-2. CLASSIFICATION OF NERVE FIBERS
Sympathetic Nervous System
The efferent SNS is referred to as the thoracolumbar nervous system. Figure 15-1 demonstrates the distribution of the SNS and its innervation of visceral organs. The preganglionic fibers of the SNS (thoracolumbar division) originate in the intermediolateral gray column of the 12 thoracic (T1-12) and the first three lumbar segments (L1-3) of the spinal cord. The myelinated axons of these nerve cells leave the spinal cord with the motor fibers to form the white (myelinated) communicating rami (Fig. 15-3). The rami enter one of the paired 22 sympathetic ganglia at their respective segmental levels. Upon entering the paravertebral ganglia of the lateral sympathetic chain, the preganglionic fiber may follow one of three courses: (1) synapse with postganglionic fibers in ganglia at the level of exit, (2) course upward or downward in the trunk of the SNS chain to synapse in ganglia at other levels, or (3) track for variable distances through the sympathetic chain and exit without synapsing to terminate in an outlying, unpaired, SNS collateral ganglion (Fig. 15-3). The adrenal gland is an exception to the rule. Preganglionic fibers pass directly into the adrenal medulla without synapsing in a ganglion (Fig. 15-2). The cells of the medulla are derived from neuronal tissue and are analogous to postganglionic neurons.
FIGURE 15-3. The spinal reflex arc of the somatic nerves is shown on the left. The different arrangements of neurons in the sympathetic system are shown on the right. Preganglionic fibers coming out through white rami may make synaptic connections following one of three courses: (1) synapse in ganglia at the level of exit, (2) course up or down the sympathetic chain to synapse at another level, or (3) exit the chain without synapsing to an outlying collateral ganglion.
The sympathetic postganglionic neuronal cell bodies are located in ganglia of the paired lateral SNS chain or unpaired collateral ganglia in more peripheral plexuses. Collateral ganglia, such as the celiac and inferior mesenteric ganglia (plexus), are formed by the convergence of preganglionic fibers with many postganglionic neuronal bodies. SNS ganglia are almost always located closer to the spinal cord than to the organs they innervate. The sympathetic postganglionic neurons can therefore originate in either the paired lateral paravertebral SNS ganglia or one of the unpaired collateral plexus. The unmyelinated postganglionic fibers then proceed from the ganglia to terminate within the organs they innervate. Many of the postganglionic fibers pass from the lateral SNS chain back into the spinal nerves, forming the gray (unmyelinated) communicating rami at all levels of the spinal cord (Fig. 15-2). They are distributed distally to sweat glands, pilomotor muscle, and blood vessels of the skin and muscle. These nerves are unmyelinated C type fibers (Table 15-2) and are carried within the somatic nerves. Approximately 8% of the fibers in the average somatic nerve are sympathetic.
The first four or five thoracic spinal segments generate preganglionic fibers that ascend in the neck to form three special paired ganglia. These are the superior cervical, middle cervical, and cervicothoracic ganglia. The last is known as the stellate ganglion and is actually formed by the fusion of the inferior cervical and first thoracic SNS ganglia. These ganglia provide sympathetic innervation of the head, neck, upper extremities, heart, and lungs. Afferent pain fibers also travel with these nerves, accounting for chest, neck, or upper extremity pain with myocardial ischemia.
Activation of the SNS produces a diffuse physiologic response (mass reflex) rather than discrete effects. SNS postganglionic neurons outnumber the preganglionic neurons in an average ratio of 20:1 to 30:1.2 One preganglionic fiber influences a larger number of postganglionic neurons, which are dispersed to many organs.
Parasympathetic Nervous System
The PNS, like the SNS, has both preganglionic and postganglionic neurons. The preganglionic cell bodies originate in the brain stem and sacral segments of the spinal cord. PNS preganglionic fibers are found in cranial nerves III (oculomotor), VII (facial), IX (glossopharyngeal), and X (vagus). The sacral outflow originates in the intermediolateral gray horns of the second, third, and fourth sacral nerves. Figure 15-1 shows the distribution of the PNS division and its innervation of visceral organs.
The vagus (cranial nerve X) nerve has the most extensive distribution of all the PNS, accounting for more than 75% of PNS activity. The paired vagus nerves supply PNS innervation to the heart, lungs, esophagus, stomach, small intestine, proximal half of the colon, liver, gallbladder, pancreas, and upper portions of the ureters. The sacral fibers form the pelvic visceral nerves, or nervi erigentes. These nerves supply the remainder of the viscera that are not innervated by the vagus. They supply the descending colon, rectum, uterus, bladder, and lower portions of the ureters and are primarily concerned with emptying. Various sexual reactions are also governed by the sacral PNS. The PNS is responsible for penile erection, but SNS stimulation governs ejaculation.
In contrast to the SNS division, PNS preganglionic fibers pass directly to the organ that is innervated. The postganglionic cell bodies are situated near or within the innervated viscera and generally are not visible. The proximity of PNS ganglia to or within the viscera provides a limited distribution of postganglionic fibers. The ratio of postganglionic to preganglionic fibers in many organs appears to be 1:1 to 3:1 compared with the 20:1 found in the SNS system. Auerbach’s plexus in the distal colon is the exception, with a ratio of 8,000:1. The fact that PNS preganglionic fibers synapse with only a few postganglionic neurons is consistent with the discrete and limited effect of PNS function. For example, vagal bradycardia can occur without a concomitant change in intestinal motility or salivation. Mass reflex action is not a characteristic of the PNS. The effects of organ response to PNS stimulation are outlined in Table 15-1.
Autonomic Innervation
Heart
The heart is well supplied by the SNS and PNS. These nerves affect cardiac pump function in three ways: (1) by changing the rate (chronotropism), (2) by changing the strength of contraction (inotropism), and (3) by modulating coronary blood flow. The PNS cardiac vagal fibers approach the stellate ganglia and then join the efferent cardiac SNS fibers; therefore, the vagus nerve to the heart and lungs is a mixed nerve containing both PNS and SNS efferent fibers. The PNS fibers are distributed mainly to the sinoatrial and atrioventricular (AV) nodes and to a lesser extent to the atria. There is little or no distribution to the ventricles. Therefore, the main effect of vagal cardiac stimulation to the heart is chronotropic. Vagal stimulation decreases the rate of sinoatrial node discharge and decreases excitability of the AV junctional fibers, slowing impulse conduction to the ventricles. A strong vagal discharge can completely arrest sinoatrial node firing and block impulse conduction to the ventricles.3
The physiologic importance of the PNS on myocardial contractility is not as well understood as that of the SNS. Cholinergic blockade can double the heart rate (HR) without altering contractility of the left ventricle. Vagal stimulation of the heart can reduce left ventricular maximum rate of tension development (dP/dT) and decrease contractile force by as much as 10% to 20%. However, PNS stimulation is relatively unimportant in this regard compared with its predominant effect on HR. The SNS has the same supraventricular distribution as the PNS, but with stronger representation to the ventricles. SNS efferents to the myocardium funnel through the paired stellate ganglia. The right stellate ganglion distributes primarily to the anterior epicardial surface and the interventricular septum. Right stellate stimulation decreases systolic duration and increases HR. The left stellate ganglion supplies the posterior and lateral surfaces of both ventricles. Left stellate stimulation increases mean arterial pressure and left ventricular contractility without causing a substantial change in HR. Normal SNS tone maintains contractility approximately 20% above that in the absence of any SNS stimulation.4 Therefore, the dominant effect of the ANS on myocardial contractility is mediated primarily through the SNS. Intrinsic mechanisms of the myocardium, however, can maintain circulation quite well without the ANS, as evidenced by the success of cardiac transplants (see Chapter 51 Transplant anesthesia). The heart and ANS are in perfect symbiosis. ANS via its components imprints the cardiac electrophysiology by potentially inducing significant dysrhythmias or electrocardiographic abnormalities, which in the end may lead to global cardiac dysfunction. The precise role of the ANS is unknown, specifically if it is an active component or just an accompaniment. Future research interests concern the modification of the autonomic cardiac innervation through pharmacology or using alternative approaches.5 Early investigations, performed in anesthetized, open-chest animals, demonstrated that cardiac ANS nerves exert only slight effects on the coronary vascular bed; however, more recent studies on chronically instrumented, intact, conscious animals show considerable evidence for a strong SNS regulation of the small coronary resistance and larger conductance vessels6,7 (see below Adrenergic Receptors).
Different segments of the coronary arterial tree react differently to various stimuli and drugs. Normally, the large conductance vessels contribute little to overall coronary vascular resistance (see Chapter 10 Cardiac Anatomy and Physiology). Fluctuations in resistance reflect changes in lumen size of the small, precapillary vessels. Blood flow through the resistance vessels is regulated primarily by the local metabolic requirements of the myocardium. The larger conductance vessels, however, can constrict markedly due to neurogenic stimulation. Neurogenic influence also assumes a greater role in the resistance vessels when they become hypoxic and lose autoregulation.
Peripheral Circulation
The SNS nerves are by far the most important regulators of the peripheral circulation. The PNS nerves play only a minor role in this regard. The PNS dilates vessels, but only in limited areas such as the genitalia. SNS stimulation produces both vasodilation and vasoconstriction, with vasoconstrictor effects predominating. The SNS effect on the vascular bed is determined by the type of receptors on which the SNS fiber terminates (see Adrenergic Receptors below). SNS constrictor receptors are distributed to all segments of the circulation. Blood vessels in the skin, kidneys, spleen, and mesentery have an extensive SNS distribution, whereas those in the heart, brain, and muscle have less SNS innervation.
Basal vasomotor tone is maintained by impulses from the lateral portion of the vasomotor center in the medulla oblongata that continually transmits impulses through the SNS, maintaining partial arteriolar and venular constriction. Circulating epinephrine (EPI) from the adrenal medulla has additive effects. This basal ANS tone maintains arteriolar constriction at an intermediate diameter. The arteriole, therefore, has the potential for either further constriction or dilation. If the basal tones were not present, the SNS could only affect vasoconstriction and not vasodilation.8 The SNS tone in the venules produces little resistance to flow compared with the arterioles and the arteries. The importance of SNS stimulation of veins is to reduce or increase their capacity. By functioning as a reservoir for approximately 80% of the total blood volume, small changes in venous capacitance produce large changes in venous return and, thus, cardiac preload.
Lungs
The lungs are innervated by both the SNS and PNS. Postganglionic SNS fibers from the upper thoracic ganglia (stellate) pass to the lungs to innervate the smooth muscles of the bronchi and pulmonary blood vessels. PNS innervation of these structures is via the vagus nerve. SNS stimulation produces bronchodilation and pulmonary vasoconstriction.9 Little else has been proven conclusively about the vasomotor control of the pulmonary vessels other than that they adjust to accommodate the output of the right ventricle. The effect of stimulation of the pulmonary SNS nerves on pulmonary vascular resistance is not ideal but may be important in maintaining hemodynamic stability during stress and exercise by balancing right and left ventricular output. Stimulation of the vagus nerve produces almost no vasodilation of the pulmonary circulation. Hypoxic pulmonary vasoconstriction is a local phenomenon capable of providing a faster adjustment to the organism needs.
Both the SNS and the vagus nerve provide active bronchomotor control. SNS stimulation causes bronchodilation whereas vagal stimulation produces constriction. PNS stimulation may also increase secretions of the bronchial glands. Vagal receptor endings in the alveolar ducts also play an important role in the reflex regulation of the ventilation cycle. The lung has important non-ventilatory activity as well. It serves as a metabolic organ that removes local mediators such as norepinephrine (NE) from the circulation and converts others, such as angiotensin 1, to active compounds10 (see below Interaction with Other Regulatory Systems).
Autonomic Nervous System Transmission
Transmission of excitation across the terminal junctional sites (synaptic clefts) of the peripheral ANS occurs through the mediation of released chemicals (Fig. 15-4). Transmitters interact with receptors on the end organ to evoke a biologic response.
FIGURE 15-4. The anatomy and physiology of the terminal postganglionic fibers of sympathetic and parasympathetic fibers are similar.
The ANS can be pharmacologically subdivided by the neurotransmitter secreted at the effector cell. Pharmacologic parlance designates the SNS and PNS as adrenergic and cholinergic, respectively. The terminals of the PNS postganglionic fibers release acetylcholine (ACh). With the exception of sweat glands, NE is the principal neurotransmitter released at the terminals of the sympathetic postganglionic fibers (see Fig. 15-2). Co-transmission of adenosine triphosphate (ATP), neuropeptide Y (NPY), and NE has been demonstrated at vascular sympathetic nerve terminals in a number of different tissues including muscle, intestine, kidney, and skin (see below SNS Neurotransmission). The preganglionic neurons of both systems secrete ACh.
The terminations of the postganglionic fibers of both ANS subdivisions are anatomically and physiologically similar. The terminations are characterized by multiple branchings called terminal effector plexuses, or reticulae. These filaments surround the elements of the effector unit “like a mesh stocking”.8 Thus, one SNS postganglionic neuron, for example, can innervate ∼25,000 effector cells, for example, vascular smooth muscle. The terminal filaments end in presynaptic enlargements called varicosities. Each varicosity contains vesicles, ∼500 μm in diameter, in which the neurotransmitters are stored (Fig. 15-4). The rate of synthesis depends on the level of ANS activity and is regulated by local feedback. The distance between the varicosity and the effector cell (synaptic or junctional cleft) varies from 100 μm in ganglia and arterioles to as much as 20,000 μm in large arteries. The time for diffusion is directly proportional to the width of the synaptic gap. Depolarization on the nerve releases the vesicular contents into the synaptic cleft by exocytosis.
Parasympathetic Nervous System Transmission
Synthesis
ACh is considered the primary neurotransmitter of the PNS. ACh is formed in the presynaptic terminal by acetylation of choline with acetyl coenzyme A. This step is catalyzed by choline acetyl transferase (Fig. 15-5). ACh is then stored in a concentrated form in presynaptic vesicles. A continual release of small amounts of ACh, called quanta, occurs during the resting state. Each quantum results in small changes in the electrical potential of the synaptic end plate without producing depolarization. These are known as miniature end-plate potentials. Arrival of an action potential causes a synchronous release of hundreds of quanta, resulting in depolarization of the end plate. Release of ACh from the vesicles is dependent on influx of calcium (Ca2+) from the interstitial space. ACh is not reused like NE; therefore, it must be synthesized constantly.
FIGURE 15-5. Synthesis and metabolism of acetylcholine.
Metabolism
The ability of a receptor to modulate function of an effector organ is dependent upon rapid recovery to its baseline state after stimulation. For this to occur, the neurotransmitter must be quickly removed from the vicinity of the receptor. ACh removal occurs by rapid hydrolysis by acetylcholinesterase (Fig. 15-5). This enzyme is found in neurons, at the neuromuscular junction, and in various other tissues of the body. A similar enzyme, pseudocholinesterase or plasma cholinesterase is also found throughout the body but only to a limited extent in neural tissue. It does not appear to be physiologically important in termination of the action of ACh. Both acetylcholinesterase and pseudocholinesterase hydrolyze ACh as well as other esters (such as the ester-type local anesthetics), and they may be distinguished by specific biochemical tests.3
Sympathetic Nervous System Transmission
Traditionally, the catecholamines EPI and NE are considered the main mediators of peripheral SNS activity. NE is released from localized presynaptic vesicles of nearly all postganglionic sympathetic nerves. Vascular SNS nerve terminals, however, also release ATP. Thus, ATP and NE are co-neurotransmitters. They are released directly to there site of action. Their postjunctional effects appear to be synergistic in tissues.
The SNS fibers ending in the adrenal medulla are preganglionic, and ACh is the neurotransmitter (see Fig. 15-2). It interacts with the chromaffin cells in the adrenal medulla, causing release of EPI and NE. The chromaffin cells take the place of the postganglionic neurons. Stimulation of the sympathetic nerves innervating the adrenal medulla, however, causes the release of large quantities of a mixture of EPI and NE into the circulation. The greater portion of this hormonal surge is normally EPI. EPI and NE, when released into the circulation, are classified as hormones in that they are synthesized, stored, and released from the adrenal medulla to act at distant sites.
Hormonal EPI and NE have essentially the same effects on effector cells as those caused by local direct sympathetic stimulation. The hormonal effects, although brief, last about 10 times as long as those caused by direct stimulation. EPI has a greater metabolic effect than NE. It can increase the metabolic rate of the body as much as 100%. It also increases glycogenolysis in the liver and muscle with glucose release into the blood. These functions are all necessary to prepare the body for fight or flight.
Catecholamines: The First Messenger
A catecholamine is any compound with a catechol nucleus (a benzene ring with two adjacent hydroxyl groups) and an amine-containing side chain. The chemical configuration of five of the more common catecholamines in clinical use is demonstrated in Figure 15-6. The endogenous catecholamines in humans are dopamine (DA), NE, and EPI. Dopamine is a neurotransmitter present in the CNS, primarily involved in coordinating motor activity in the brain. It is the precursor of NE. NE is synthesized and stored in nerve endings of postganglionic SNS neurons. It is also synthesized in the adrenal medulla and is the chemical precursor of EPI. Stored EPI is located chiefly in chromaffin cells of the adrenal medulla. About 80% to 85% of the catecholamine content of the adrenal medulla is EPI and 15% to 20% is NE. The brain contains both noradrenergic and dopaminergic receptors, but circulating catecholamines do not cross the blood–brain barrier. The catecholamines present in the brain are synthesized there.
FIGURE 15-6. The chemical configurations of three endogenous catecholamines are compared with those of three synthetic catecholamines. Sympathomimetic drugs differ in their hemodynamic effects largely because of differences in substitution of the amine group on the catechol nucleus.
Catecholamines are often referred to as adrenergic drugs because their effector actions are mediated through receptors specific for the SNS. Sympathomimetics can activate these same receptors because of their structural similarity. For example, clonidine is an α2-receptor agonist that does not possess a catechol nucleus and even has two ring systems that are aplanar to each other. However, clonidine enjoys a remarkable spatial similarity to NE that allows it to activate the a receptor. Drugs that produce sympathetic-like effects but lack basic catecholamine structure are defined as sympathomimetics. All clinically useful catecholamines are sympathomimetics, but not all sympathomimetics are catecholamines. The effects of endogenous or synthetic catecholamines on adrenergic receptors can be direct or indirect. Indirect-acting catecholamines (i.e., ephedrine) have little intrinsic effect on adrenergic receptors but produce their effects by stimulating release of the stored neurotransmitter from SNS nerve terminals. Some synthetic and endogenous catecholamines stimulate adrenergic receptor sites directly (e.g., phenylephirine), whereas others have a mixed mode of action. The actions of direct-acting catecholamines are independent of endogenous NE stores; however, the indirect-acting catecholamines are entirely dependent on adequate neuronal stores of endogenous NE.
Synthesis
The main site of NE synthesis is in or near the postganglionic nerve endings. Some synthesis does occur in vesicles near the cell body that pass to the nerve endings. Phenylalanine or tyrosine is taken up into the axoplasm of the nerve terminal and modified into either NE or EPI. Figure 15-7 demonstrates this synthesis cascade. Tyrosine hydroxylase catalyzes the conversion of tyrosine to dihydroxyphenylalanine. This is the rate-limiting step at which NE synthesis is controlled through feedback inhibition. Dopamine (DA) synthesis occurs in the cytoplasm of the neuron. The vesicles of peripheral postganglionic neurons contain the enzyme dopamine-b-hydroxylase, which converts dopamine to NE. The adrenal medulla additionally contains phenylethanolamine-N-methyltransferase, which converts NE to EPI. This reaction takes place outside the medullary vesicles, and the newly formed EPI then enters the vesicle for storage (Fig. 15-8). All the endogenous catecholamines are stored in presynaptic vesicles and released on arrival of an action potential. Excitation–secretion coupling in sympathetic neurons is Ca2+-dependent.
FIGURE 15-7. Schematic of the synthesis of catecholamines. The conversion of tyrosine to DOPA by tyrosine hydroxylase is inhibited by increased NE synthesis. Epinephrine is shown in these steps but is primarily synthesized in the adrenal medulla. DOPA, dihydroxyphenylalanine.
FIGURE 15-8. Schematic of the synthesis and disposition of NE in adrenergic neurotransmission. (1) Synthesis and storage in neuronal vesicles; (2) Action potential permits calcium entry with (3) exocytosis of NE into synaptic gap. (4) Released NE reacts with receptors on effector cell. NE (5) may react with presynaptic α-2 receptor to inhibit further NE release or with presynaptic β-receptor to enhance reuptake of NE (6) (uptake 1). Extraneuronal uptake (uptake 2) absorbs NE into effector cell (7) with overflow occurring systemically (8). MAO, monoamine oxidase; COMT, catechol-O-methyltransferase; Tyr, tyrosine; DOPA, dihydroxyphenylalanine; NE, norepinephrine.
Regulation
Increased SNS nervous activity, as in congestive heart failure or chronic stress, stimulates the synthesis of catecholamines. Glucocorticoids from the adrenal cortex stimulate an increase in phenylethanolamine-N-methyltransferase that methylates NE to EPI.
The release of NE is dependent upon depolarization of the nerve and an increase in calcium ion permeability. This release is inhibited by colchicine and prostaglandin E2, suggesting a contractile mechanism. NE inhibits its own release by stimulating presynaptic (prejunctional) α2 receptors. Phenoxybenzamine and phentolamine, α-receptor antagonists, increase the release of NE by blocking inhibitory presynaptic α2 receptors (Fig. 15-9). Other receptors are also important in NE regulation (see below Other Receptors).
FIGURE 15-9. This schematic demonstrates just a few of the presynaptic adrenergic receptors thought to exist. Agonist and antagonist drugs are clinically available for these receptors (see Table 15-5). The α-2 receptors serve as a negative feedback mechanism whereby NE stimulation inhibits its own release. Presynaptic β-stimulation increases NE uptake, augmenting its availability. Presynaptic muscarinic (MUSC) receptors respond to ACh diffusing from nearby cholinergic terminals. They inhibit NE release and can be blocked by atropine. NE, norepinephrine.
Inactivation
The catecholamines are removed from the synaptic cleft by three mechanisms (Fig. 15-8). These are reuptake into the presynaptic terminals, extraneuronal uptake, and diffusion. Termination of NE action at the effector site is almost entirely by reuptake of NE into the terminals of the presynaptic neuron. This is an active, energy-requiring, and temperature-dependent process. The reuptake of NE in the presynaptic terminals is also a stereospecific process. Structurally similar compounds (guanethidine, metaraminol) may enter the vesicles and displace the neurotransmitter. Tricyclic antidepressants and cocaine inhibit the reuptake of NE, resulting in high synaptic NE concentrations and accentuated receptor response. In addition, evidence suggests that NE reuptake is mediated by a presynaptic β-adrenergic mechanism because β-blockade causes marked elevations of EPI and NE11 (see Figs. 15-8 and 15-9). Extraneuronal uptake is a minor pathway for inactivating NE. Effector cells and other extraneuronal tissues take up NE. The NE that is taken up by the extraneuronal tissue is metabolized by monoamine oxidase (MAO) and by catechol-O-methyltransferase (COMT) to form vanillylmandelic acid. The minute amount of catecholamine that escapes these two mechanisms diffuses into the circulation, where it is metabolized by the liver and kidney. The same enzymes inactivate EPI. Reuptake is the predominant pathway for inactivation of the endogenous catecholamines, while metabolism by the liver and kidney is the predominant pathway for catecholamines given exogenously. This accounts for the longer duration of action of the exogenous catecholamines than that noted at the local synapse.
The final metabolic product of the catecholamines is vanillylmandelic acid. Vanillylmandelic acid constitutes the major metabolite (80% to 90%) of NE found in the urine. Less than 5% of released NE appears unchanged in the urine. The metabolic products excreted in the urine provide a gross estimate of SNS activity and can facilitate the clinical diagnosis of pheochromocytoma (see Chapter 46, Endocrine Function).
RECEPTORS
An agonist is a substance that interacts with a receptor to evoke a biologic response. ACh, NE, EPI, DA, and ATP are the major agonists of the ANS. An antagonist is a substance that interferes with the evocation of a response at a receptor site by an agonist. Receptors are therefore target sites that lead to a response by the effector cell when activated by an agonist. Receptors are protein macromolecules and are located in the plasma membrane. Several thousand receptors have been demonstrated in a single cell. The enormity of this network becomes apparent when one considers that ∼25,000 single cells can be innervated by a single neuron.
Cholinergic Receptors
ACh is the neurotransmitter for three distinct classes of receptors. These receptors can be differentiated by their anatomic location and their affinity for various agonists and antagonists. ACh mediates the “first messenger” function of transmitting impulses within the PNS, the ganglia of the SNS, and the neuroeffector junction of striated, voluntary muscle (see Fig. 15-2). Cholinergic receptors are further subdivided into muscarinic and nicotinic receptors because muscarine and nicotine stimulate them selectively. However, both muscarinic and nicotinic receptors respond to ACh (see below Cholinergic Drugs). Muscarine activates cholinergic receptors at the postganglionic PNS junctions of cardiac and smooth muscle. Muscarinic stimulation is characterized by bradycardia, decreased inotropism, bronchoconstriction, miosis, salivation, gastrointestinal hypermotility, and increased gastric acid secretion (see Table 15-1). Muscarinic receptors can be blocked by atropine without effect on nicotinic receptors (see below Cholinergic Drugs). Muscarinic receptors are known to exist in sites other than PNS postganglionic junctions. They are found on the presynaptic membrane of sympathetic nerve terminals in the myocardium, coronary vessels, and peripheral vasculature (Fig. 15-9). These are referred to as adrenergic muscarinic receptors because of their location; however, ACh stimulates them also. Stimulation of these receptors inhibits release of NE in a manner similar to α2-receptor stimulation. Muscarinic blockade removes the inhibition of NE release, augmenting SNS activity. Atropine, the prototypical muscarinic blocker, may produce sympathomimetic activity in this manner as well as vagal blockade. Neuromuscular blocking drugs that cause tachycardia are thought to have a similar mechanism of action. ACh acting on presynaptic adrenergic muscarinic receptors is a potent inhibitor of NE release.11 The prejunctional muscarinic receptor may play an important physiologic role because several autonomically innervated tissues (e.g., the heart) possess ANS plexuses in which the SNS and PNS nerve terminals are closely associated. In these plexuses, ACh, released from the nearby PNS nerve terminals (vagus nerve), can inhibit NE release by activation of presynaptic adrenergic muscarinic receptors (Fig. 15-9).
Nicotinic receptors are found at the synaptic junctions of both SNS and PNS ganglia. Because both junctions are cholinergic, ACh or ACh-like substances such as nicotine will excite postganglionic fibers of both systems (see Fig. 15-2). Low doses of nicotine produce stimulation of ANS ganglia whereas high doses produce blockade. This dualism is referred to as the nicotinic effect (see below Ganglionic Drugs). Nicotinic stimulation of the SNS ganglia produces hypertension and tachycardia by causing the release of EPI and NE from the adrenal medulla. Adrenal hormone release is mediated by ACh in the chromaffin cells, which are analogous to postganglionic neurons (see Fig. 15-2). A further increase in nicotine concentration produces hypotension and neuromuscular weakness, as it becomes a ganglionic blocker. The cholinergic neuroeffector junction of skeletal muscle also contains nicotinic receptors, although they are not identical to the nicotinic receptors in ANS ganglia.
Adrenergic Receptors
The adrenergic receptors are termed adrenergic or noradrenergic, depending on their responsiveness to EPI or NE. The dissimilarities of these two drugs led Ahlquist in 1948 to propose two types of opposing adrenergic receptors, termed alpha (α) and beta (β). The development of new agonists and antagonists with relatively selective activity allowed subdivision the β-receptors into β1 and β2. α-receptors were subsequently divided into α1 and α2. These were later further subdivided using molecular cloning. The sympathomimetic adrenergic drugs in current use differ from one another in their effects largely because of differences in substitution on the amine group, which influences the relative α or β effect (see Fig. 15-6).
Another major peripheral adrenergic receptor specific for dopamine is termed the dopaminergic (DA) receptor. Further studies have revealed not only subsets of the α and β receptors but also the DA receptor. These DA receptors have been identified in the CNS and in renal, mesenteric, and coronary vessels. The physiologic importance of these receptors is a matter of controversy because there are no identifiable peripheral DA neurons. Dopamine measured in the circulation is assumed to result from spillover from the brain.
The function of dopamine in the CNS has long been known, but the peripheral dopamine receptor has been elucidated only within the past 25 years. The presence of the peripheral DA receptor was obscured because dopamine does not affect the DA receptor exclusively. It also stimulates α and β receptors in a dose-related manner. However, DA receptors function independently of α or β blockade and are modified by DA antagonists such as haloperidol, droperidol, and phenothiazines. Thus, there is a necessity for the addition of the DA receptor and its subsets (DA1 and DA2).
The distribution of adrenergic receptors in organs and tissues is not uniform and their function differs not only by their location but also in their numbers and/or distribution. Adrenergic receptors are found in two loci in the sympathetic neuroeffector junction. They are found in both the presynaptic (prejunctional) and postsynaptic (postjunctional) sites as well as extrasynaptic sites (Fig. 15-10). Table 15-3 is a review of the function and synaptic location of some of the clinically important receptors and their subtypes.
FIGURE 15-10. Location of several known adrenergic receptors. The presynaptic α-2 and DA receptors serve as a negative feedback mechanism, whereby stimulation of NE inhibits its own release. Presynaptic β-2 stimulation increases NE uptake, augmenting its availability. Postsynaptic α-2 and β-2 receptors are extrasynaptic and are considered non-innervated hormonal receptors. DA, dopamine; NE, norepinephrine.
TABLE 15-3. ADRENERGIC RECEPTORS: ORDER OF POTENCY OF AGONISTS AND ANTAGONISTS
Alpha-adrenergic Receptors
The alpha-adrenergic (α) receptors have been further subdivided into two clinically important classes, α1 and α2. This classification is based on their response to the α-antagonists yohimbine and prazosin. Prazosin is a more potent antagonist of α1 receptors, whereas α2 receptors are more sensitive to yohimbine. Recently, pharmacologic experiments have demonstrated the existence of two subtypes within the α1 group, namely α1A and α1B, and at least two subtypes within the α2, group respectively α2A, and α2B. The importance of these subsets is still emerging, with evidence that the spleen and liver contain mainly α1B receptors, and the heart, neocortex, kidney, vas deferens, and hippocampus contain equal amounts of α1A and α1B receptors. The α1-adrenergic receptors are found in the smooth muscle cells of the peripheral vasculature coronary arteries, skin, uterus, intestinal mucosa, and splanchnic beds12 (see Table 15-4). The α1 receptors serve as postsynaptic activators of vascular and intestinal smooth muscle as well as of endocrine glands. Their activation results in either decreased or increased tone, depending upon the effector organ. The response in resistance and capacitance vessels is constriction, whereas in the intestinal tract it is relaxation. There is now a large body of evidence documenting the presence of postjunctional α1 adrenoreceptors in the mammalian heart. α1-adrenergic receptors have been shown to have a positive inotropic effect on cardiac tissues in most mammals studied, including humans. Experimental work strongly supports the concept that enhanced myocardial α1 responsiveness plays a primary role in the genesis of malignant arrhythmias induced by catecholamines during myocardial ischemia and reperfusion. Drugs possessing potent α1-antagonist activity such as prazosin and phentolamine provide significant antiarrhythmic activity. The clinical mechanism and significance of these findings are not yet clear. However, there is no doubt that α1-adrenergic antagonists prevent catecholamine-induced ventricular arrhythmias.13 In contrast, studies of the effects of β-antagonists in experimental and clinical myocardial infarction have provided conflicting results.
TABLE 15-4. ADRENERGIC RECEPTORS
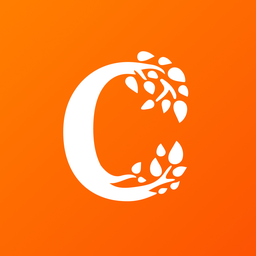
Full access? Get Clinical Tree
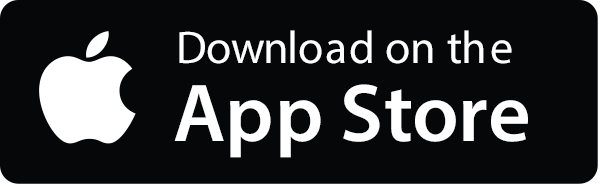
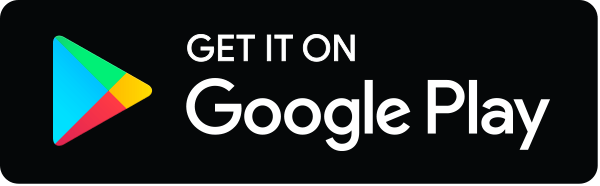