Kenneth M. Brady1, Chandra Ramamoorthy2, R. Blaine Easley3, and Dean B. Andropoulos3 1 Division of Cardiac Anesthesia Regenstein Cardiac Care Unit, Gracias Family Professor in Cardiac Critical Care, Department of Anesthesiology and Pediatrics, Lurie Children’s Hospital of Chicago, Northwestern University Feinberg School of Medicine, Chicago, IL, USA 2 Department of Anesthesiology, Perioperative and Pain Medicine, Lucille Packard Children’s Hospital, Stanford University School of Medicine, Stanford, CA, USA 3 Department of Anesthesiology, Perioperative and Pain Medicine, Department of Anesthesiology, Baylor College of Medicine, Texas Children’s Hospital, Houston, TX, USA Operative management of the child with congenital heart disease (CHD) was largely refined to its current state in the decades prior to the year 2000, during which operative mortality improved substantially. Data from the metropolitan Atlanta congenital defects program showed a profound increase in 1‐year survival of the most critical heart defects, including hypoplastic left heart syndrome (HLHS), truncus arteriosus, pulmonary atresia, tricuspid atresia, and Tetralogy of Fallot from 67% (1979–1993) to 83% (1994–2005) [1]. Predictably, the increased expectation of survival is accompanied by willingness to perform surgery on increasingly complex patients. A multi‐institution comparison of survival rates by case complexity using the Risk Adjustment for Congenital Heart Surgery (RACHS) showed a shift of fewer RACHS category 1 cases (low complexity) and more RACHS category 3, 4, and 6 cases (higher complexity) between 1996 and 2004. Further, mortality of RACHS category 6 (predominantly Norwood stage I palliation for HLHS) cases decreased from 48% in 1996 to 17% in 2004 [2]. The most common neonatal operations in the Society of Thoracic Surgeons’ Congenital Heart Surgery Database are Norwood stage 1 palliation at 10.2% and arterial switch operation without ventricular septal defect (VSD) at 6.9%, and the surgical mortality of these lesions has decreased to 13.8%, and 2.0%, respectively, as of 2019 [3]. Despite these remarkable gains, survivors of complex CHD are at risk of having a characteristic array of neurodevelopmental deficits. These include poor impulse control, attention deficit/hyperactivity, mild language and cognitive deficits, and limited executive functioning ability [4–8]. Often appearing outwardly normal, children with such deficits perform poorly in academics and lack employability; they suffer high rates of depression and poor quality of life [9–14]. In addition to potentially modifiable factors, the presence of genetic syndromes and increasing complexity of the cardiac lesion are associated with a higher incidence of neurodevelopmental disability (Figure 14.1) [15]. The relative role of modifiable (acute neurologic injuries) and nonmodifiable factors contributing to the neurologic abnormalities seen in patients with CHD is a subject of study and debate. This chapter, written for the anesthesiologist, has an understandable bias toward discussion of the potentially modifiable perioperative factors that have been associated with neurologic injury. The pediatric cardiac anesthesiologist should be familiar with acute, perioperatively acquired neurologic injuries in this population and proposed strategies to avoid them. Acute neurologic injury in the perioperative period has been quantified using magnetic resonance imaging (MRI) technology. Preoperative neurologic injuries visible by MRI occur with an incidence between 20 and 40% in newborns with critical heart defects [8, 16–18]. After surgery, 35–75% of patients will have a new lesion [19]. Despite differences in nomenclature of MRI findings, it is clear that the preponderance of new injuries seen after cardiac surgery in the infant occurs in the white matter (see Figure 14.2) [16, 17, 19–23]. Most studies further specified the preponderant white matter lesion as periventricular leukomalacia, an injury that has been extensively described in preterm infants exposed to extrauterine life (see Figure 14.3). In the preterm population, periventricular leukomalacia is associated with reduction in brain growth and neurodevelopmental impairments with deficits similar to those described in children with critical heart lesions [24, 25]. Current understanding of the pathogenesis of preterm periventricular leukomalacia posits that the preterm brain is susceptible to ischemia due to an incomplete redundancy of penetrating arterioles in the deep white matter, combined with a population of developing oligodendrocyte precursors with enhanced ischemic sensitivity [26–28]. This pattern of injury in the patient with CHD suggests that oxygen deprivation is the leading cause of intraoperative injury, not embolic or hemorrhagic injury as is more commonly seen in adults. Figure 14.1 Schematic presentation of neurodevelopmental abnormalities in children with congenital heart disease (CHD). Children with milder forms of CHD (e.g., ventricular septal defect without an associated genetic syndrome) have a low incidence, and more than mild abnormalities are rare. Increasingly complex forms of CHD (e.g., transposition or total anomalous pulmonary venous connection) are associated with increasing risk of neurodevelopmental deficits. Only the minority of children with very complex CHD (e.g., functionally univentricular heart, hypoplastic left heart syndrome) are completely normal in all respects. CHD associated with chromosomal abnormalities (e.g., Down’s and DiGeorge syndromes) or multiple congenital anomalies (VACTERL, CHARGE associations) are nearly always associated with neurodevelopmental abnormalities, in many cases severe. (Source: Wernovsky [15]. Reproduced with permission from Cambridge University Press.) It has been assumed that improvements in hemodynamic management during cardiac surgery have the potential to mitigate neurologic injuries in children who require cardiopulmonary bypass (CPB). However, the management of CPB for infants and children has been challenging to study clinically due to wide practice variability and lack of intercenter consensus. Pump prime, flow rate, cardioplegia solutions and administration intervals, vasodilator therapy, steroid use, cooling and rewarming practices, blood gas management, hematocrit goals, electrolyte control, ultrafiltration techniques, and the use of deep hypothermic circulatory arrest (DHCA) are all integral aspects of a complex practice that forms the protocol at a given institution. Each of these can impact cerebral perfusion, but no standardization exists for any of these parameters. Therefore, understanding the consequence of altering a single aspect of bypass care is unclear, and demonstrating the optimal combination of bypass parameters is prohibitively difficult. Figure 14.2 The incidence of preexisting and new postoperative neurologic injuries diagnosed by brain magnetic resonance imaging (MRI) observed in neonates subjected to cardiopulmonary bypass. The findings are compiled from six reports, seven centers, and 400 subjects. Median and range are shown with on outlier. WMI, white matter injury. Data are from references [16–23]. Due to the pressing need to improve neurocognitive outcome and poor guidance afforded by evidence‐based approaches to bypass management, effort and expense have been directed toward developing perioperative neuromonitoring strategies for children who require cardiac surgery and CPB. The monitoring technologies described in this chapter have been in routine use for more than a decade, but there is no universal consensus regarding their usefulness or validity, and there is no standard of care neuromonitoring practice for the patient with CHD. This chapter reviews cerebrovascular physiology and the impact of CPB on cerebral hemodynamics, available neuromonitoring modalities, and proposed strategies for improving neurodevelopmental outcomes after congenital heart surgery. Figure 14.3 (A) Preoperative sagittal T1‐weighted magnetic resonance image (MRI) of a 35‐week gestational age infant with hypoplastic left heart syndrome. Significant white matter injury (WMI) is present in the periventricular areas (arrows). (B) Preoperative axial proton‐density T2‐weighted image. Again note the extensive WMI (arrows). (C) Seven‐day postoperative T1 sagittal MRI after Norwood stage I palliation. Note the slight improvement to the WMI, but new intraparenchymal/intraventricular hemorrhage and infarction in the atrium near the body of the left lateral ventricle (arrow). (D) Proton‐density T2‐weighted image. Again note the WMI and new hemorrhage (arrow). Understanding cerebral perfusion and how it is affected by CPB is necessary to understand normal and abnormal neuromonitoring results during cardiac surgery. In health, cerebral perfusion regulation is known to be a finely tuned, multilayered servo control of several homeostatic mechanisms, each acting independently with regard to stimulus and frequency, but in concert at the point of action: mediating cerebral vascular resistance. These mechanisms include neurovascular coupling (also known as metabolic autoregulation), CO2 reactivity, pressure autoregulation, hypoxic vasodilatation, hypoglycemic vasodilatation, and the uneven action of systemic vasoconstrictors to cerebral relative to noncerebral vasculature. Physiologic changes of the cerebral vasculature during bypass were largely defined by a series of studies performed in the 1980s and 1990s. Cerebral blood flow (CBF) was measured using the Kety–Schmidt technique, and CBF velocity was measured with transcranial Doppler (TCD) in both human infant and animal model studies. These efforts have highlighted the effects of extreme hypothermia and circulatory arrest on vascular tone, CBF, and oxygen delivery and utilization within the brain. During the cooling phase of CPB, CBF is reduced, and cerebral metabolism is reduced, presumably due to neurovascular coupling, which matches CBF to metabolism. However, during deep hypothermia, cerebral metabolism is exponentially reduced, while CBF is reduced linearly with reduction in temperature [29] (see Figure 14.4). This results in a state of “luxury perfusion” during hypothermia [30–33]. As shown another way, at normothermic bypass between 35.5 °C and 37 °C, the ratio of CBF (in mL/100 g/min) to cerebral metabolic rate for oxygen consumption (CMRO2, in mL/100 g/min) is normal (20 : 1). At 30 °C, the ratio increases to 30 : 1, and at 18 °C, it increases to 75 : 1, indicating uncoupling of brain metabolism and vascular tone (see Figure 14.5) [29]. Based on the measured decrease in CMRO2 in children during deep hypothermic bypass, Greeley et al. famously estimated a duration of ischemia that could be safely tolerated between 39 and 65 minutes [31]. When rewarmed after hypothermia in the absence of circulatory arrest, there is a return to normal CBF and metabolism with normal flow/metabolism coupling. It is generally stated that cooling ablates pressure autoregulation as well as metabolic autoregulation. The two mechanisms are distinct processes that occur at distinct frequencies. Neurovascular coupling (metabolic autoregulation) is mediated by a neurovascular unit comprising an astrocyte bridge between neuronal synapses and penetrating arterioles. Neurovascular coupling is spatially specific and rapidly active, effecting vascular changes within 1 second of local neuronal activation [34]. By contrast, pressure autoregulation responds to sustained changes in cerebral perfusion pressure lasting 30 seconds or longer and causes more global changes in CBF [35]. The conclusion that hypothermia also ablates pressure autoregulation comes from two independent investigations, one using TCD and the other using the Kety–Schmidt technique with xenon washout during infant bypass [31, 33, 36]. However, in both data sets, the measurements of flow or flow velocity are taken at lower cerebral perfusion pressures than those taken at normothermia, and most of the hypothermic measurements are taken below the apparent limit of autoregulation evident in the normothermic plots [37]. Figure 14.4 Cooling results in a linear decrease in cerebral blood flow and an exponential decrease in cerebral metabolic rate for oxygen consumption (CMRO2). (Source: Adapted from Kern et al. [29].) Figure 14.5 Metabolic autoregulation is attenuated with progressive hypothermia during cardiopulmonary bypass in infants. With decreasing temperature, the ratio of cerebral blood flow (CBF) to cerebral metabolic rate for oxygen consumption (CMRO2) increases, yielding a luxury perfusion during hypothermia. (Source: Adapted from Kern et al. [29].) More recent preclinical and clinical data challenges the assumption that cooling ablates pressure autoregulation. A piglet model was used to more directly assess the relationship between deep hypothermia and pressure autoregulation. Piglets with normal arterial blood pressure demonstrated intact autoregulation, even during deep hypothermia. [38] When neonates were studied using dynamic autoregulation measurements during hypothermic bypass, the state of impaired autoregulation was accompanied, in all cases, by arterial blood pressure below the limit of autoregulation [39]. It is reasonable to conclude from this combined data that pressure autoregulation is not ablated by deep hypothermia, but rather it is impaired by the hypotensive state that frequently accompanies deep hypothermic bypass. pH‐stat management corrects blood gas values for temperature during CPB, which results in a higher arterial CO2 tension during hypothermia than is achieved with alpha‐stat blood gas management. Obviously, regardless of the measurement technique used for determining the amount of CO2 in the blood, removing less CO2 or adding CO2 to the sweep gas with the oxygenator during bypass results in a higher arterial CO2 tension. CO2 diffuses freely across the blood–brain barrier, acidifying cerebrospinal fluid, which exerts a potent cerebral vasodilatory effect. The cerebrospinal fluid slowly returns to normal pH after exposure to high CO2 levels by increased activity of carbonic anhydrase in the choroid plexus, replacing reabsorbed acidic spinal fluid with spinal fluid having a higher concentration of bicarbonate buffer. Driving CBF changes by manipulation of arterial CO2 tension is, therefore, time limited by the rate of spinal fluid absorption and production. Spinal fluid production is slower in infants than in adults, but in adults, the entire spinal fluid volume is produced three to four times per day. Not surprisingly, then, animal models have shown that sustained changes in arterial CO2 tension cause CBF changes that last approximately 1 day [40, 41]. In addition to increasing CBF, arterial hypercapnia shifts the oxyhemoglobin dissociation curve to the right, facilitating oxygen unloading [42]. Thus, in most pediatric centers, pH‐stat (hypercapnia) strategy is favored for perceived neuroprotection. In animal models of DHCA, neurologic outcome is improved when pH‐stat is used. This has been more difficult to demonstrate in human infants, although a trend toward a lower death rate, fewer seizures, and greater hemodynamic stability has been observed [43]. Long‐term neurologic follow‐up at 4 years of age has not shown a difference between pH‐stat or alpha‐stat blood gas management, and this has only been studied retrospectively in a pediatric setting [42, 44]. Traditionally, profound anemia was used during deep hypothermic CPB to offset the effect of cooling on blood viscosity. This practice, rooted in theory, was challenged by demonstration of increased neurologic injury in a piglet model of hypothermic circulatory arrest using a target hematocrit of 30% when compared with a target of 20% [45]. More convincing was a randomized study of hematocrit goals at Boston Children’s Hospital. The combined data from two trials at Boston included the neurodevelopmental outcomes of 271 infants at 1 year of age. The Psychomotor Development Index (PDI) increased linearly with hematocrit up to 23.5%. The Mental Development Index (MDI) did not exhibit an association with hematocrit. The overall conclusion of this analysis was that although a hematocrit higher than 24% improved outcome, the effects of hemodilution vary according to age and other parameters of perfusion, especially pH management, precluding delineation of a universally optimal hematocrit [46]. Hypothermia remains the cornerstone of brain protection for ischemic injury. Cerebral hyperthermia frequently develops after congenital heart surgery with CPB. The metabolic rate and oxygen consumption of the brain are raised during a period when oxygen delivery may be compromised due to hypotension. This places vulnerable watershed areas and partially injured brain tissue at risk for injury extension [47]. Bissonnette and colleagues measured temperatures in the jugular bulb, tympanic membrane, lower esophagus, and rectum during and after surgery in 15 infants and showed that the jugular bulb temperature continued to rise for at least 6 hours postoperatively [48]. The authors found that rectal temperature does not reflect cerebral temperature in the perioperative period. Other investigators further showed that nasopharyngeal and not tympanic temperature best reflects brain temperature [47]. However, Cottrell and colleagues were unable to show any association between neurocognitive performance and postoperative temperature in a cohort of 329 infants who underwent cardiac surgery [49]. In some patients, brain metabolism is not adequately suppressed during rapid cooling to deep hypothermic levels. In a study of infants undergoing cooling to 15 °C, 6 of 17 had low jugular venous bulb saturation when this temperature was achieved (87 ± 6% vs. 98 ± 1% in subjects with apparently normal cooling), suggesting ongoing oxygen consumption that outstripped delivery of oxygen to the brain [50]. There is general concern that uneven or inefficient cooling of the brain may lead to neurologic deficits. Rapid cooling times have been associated in pilot studies with developmental delay and the rare complication of choreoathetosis [51, 52]. There is little debate about the benefits of cooling during CPB, yet there is also insufficient data to suggest that any cooling strategy leads to better patient outcome [44]. Normothermic or mildly hypothermic CPB has gained popularity in recent years. Hannon and colleagues retrospectively studied 78 patients undergoing normothermic (35 °C or greater) vs. hypothermic bypass (28–34 °C) [53]. Patients were less than 2 years of age (median 7 months), and operations requiring deep hypothermia for DHCA or aortic arch reconstruction were excluded, and follow‐up was 2–3 years after surgery. For both groups, the odds ratios for neurodevelopmental delay in one or more areas ranged from 2–8 times that of a normal population. After multivariable adjustment, including for genetic conditions (Trisomy 21, DiGeorge syndrome, 22q11.2 deletion syndromes), there was no difference in the incidence of neurodevelopmental delay between the groups. In the Boston Circulatory Arrest Study (BCAS), hyperglycemia was not associated with worse neurologic outcome and does not appear to be a risk factor for infants and children [54]. Intraoperative glucose concentrations were not related to developmental scores up to 8 years of age, but low glucose concentrations were associated with subclinical seizure activity [55]. In a study of 188 neonates and infants younger than 6 months undergoing surgery with CPB, mean intensive care unit (ICU) admission glucose was 328 mg/dL, maximum was 340 mg/dL, and 89% of patients had at least one value greater than 200 mg/dL. In that cohort, hyperglycemia was not associated with lower neurodevelopment scores at 1 year of age [56]. Krueger and colleagues assessed neurodevelopmental outcomes at age 4 years in 167 infants undergoing cardiac surgery with bypass as infants [57]. Fourteen percent had hyperglycemia (>145 mg/dL) in the postoperative period, and insulin was administered if the glucose value was above 216 mg/dL. Higher postoperative glucose levels were not associated with lower neurodevelopmental scores across multiple domains. Tight glucose control is not indicated by evidentiary review in children who require CPB and is likely to be harmful in this population [44]. For infants who require reconstruction of the aortic arch, a bloodless field cannot be achieved with standard cannulation techniques. When DHCA is employed for this scenario, a profound cerebrovascular derangement occurs that persists into the rewarming and postoperative recovery periods. This cerebrovascular injury has been characterized by low CMRO2 that fails to return to baseline values, low CBF (with a normal CBF:CMRO2 ratio), and a distinctive TCD flow velocity pattern showing absent or reversed flow during diastole (see Figure 14.6) [58–61]. These derangements have been shown to be reduced in incidence by the use of low‐flow CPB instead of circulatory arrest, delayed rewarming, the use of pH‐stat blood gas management during cooling, modified ultrafiltration, thromboxane A2 antagonism, and the administration of nitric oxide donors [59, 62–66]. Although these therapies mitigate the post‐circulatory arrest physiologic derangement of the cerebral vasculature, there is no evidence that they change the incidence of periventricular leukomalacia, nor is it proven that these techniques improve the neurocognitive performance of patients with CHD. Selective cerebral perfusion (also termed regional low‐flow cerebral perfusion, antegrade cerebral perfusion, or regional cerebral perfusion) is the surgical response to concerns that circulatory arrest causes neurologic injury. A variety of techniques achieve the same goal of delivering bypass flow to the cerebral vasculature while leaving a bloodless aortic arch for surgical repair. In one version of the technique, a polytetrafluoroethylene graft is sewn into the innominate artery and subsequently cannulated for bypass, in lieu of the traditional cannulation at the aortic root. When selective perfusion is desired, the great vessels are snared, and flow is continued at a reduced rate, now entirely directed to the circle of Willis (see Figure 14.7) [67]. It seems evident that circulatory arrest and impaired neurovascular recovery after circulatory arrest would cause neurologic injury, but there is no agreement that circulatory arrest should be replaced with either low‐flow bypass or newer techniques of selective antegrade cerebral perfusion. Figure 14.6 After cardiopulmonary bypass with deep hypothermia and a period of circulatory arrest, there is an absence of cerebral blood flow during diastole. This pattern of flow velocity measured with transcranial ultrasound is indicative of high cerebrovascular resistance. (Source: Jonassen et al. [58]. Reproduced with permission from Elsevier.) Figure 14.7 Selective cerebral perfusion performed by sewing a graft to the innominate artery and snaring the great vessels. (Source: Pigula et al. [67]. Reproduced with permission from Elsevier.) For instance, neurocognitive testing of a 238‐subject cohort at 4 years of age following surgery in infancy at the Children’s Hospital of Philadelphia showed that the duration of hypothermic circulatory arrest was not associated with a poor performance in any category of the neurodevelopmental test [68]. Gaynor and colleagues argued with this result that nonmodifiable factors such as genetic anomaly, socioeconomic status, maternal education, and gestational age were overwhelmingly responsible for poor results on neurocognitive testing and that circulatory arrest is not a contributor to poor neurologic outcome. This result and interpretation contradict the most popular reading of studies related to the Boston circulatory arrest trial, which showed increased seizures and lower development scores in multiple functional domains at 1, 4, and 8 years of follow‐up in subjects who underwent circulatory arrest as a randomized intervention [4, 6, 54, 69, 70]. With later testing in the teenage years of the same cohort, differences between arrest and nonarrest groups are less apparent [71]. Although the Boston circulatory arrest trial is one of the most widely studied and cited research efforts on the topic of neurologic injury and circulatory arrest, it is difficult to compare contemporary practice with the bypass strategy from 1990, which included deliberate hemodilution to a hematocrit of 20% [72]. More recently, the “Hearts and Minds” study performed in Auckland, New Zealand, and Melbourne, Australia, found that longer periods of circulatory arrest were associated with a significant increase in the severity of periventricular WMI [22] (see Figure 14.8). In the Hearts and Minds study, many nonmodifiable characteristics were more significantly associated with WMI than the use of circulatory arrest. These included the nature of the cardiac lesion and the brain maturity score. The presence of WMI was not associated with 2‐year neurocognitive performance in that cohort. Two trials comparing circulatory arrest with selective cerebral perfusion found no difference in neurocognitive outcomes at 1 year. Visconti and colleagues studied 29 subjects with HLHS who received either selective cerebral perfusion or circulatory arrest without randomization for the Norwood procedure [73]. Goldberg and colleagues studied 77 subjects with the same condition, randomized for the two bypass strategies [74]. Neither study showed significant improvements with the selective perfusion technique, but it has been argued that neurodevelopmental scores in both groups were lower than expected. Figure 14.8 Data from the Hearts and Minds study showed that longer circulatory arrest times were associated with an increase in the severity of white matter injury. DHCA, deep hypothermic circulatory arrest. (Source: Beca et al. [22]. Reproduced with permission from Wolters Kluwer Health, Inc.) Another randomized trial of selective cerebral perfusion vs. circulatory arrest was done with 37 neonates requiring arch reconstruction. More than 70% of subjects in both groups of that study had a new MRI injury. WMI was the most common new lesion in both groups, but 33% of the subjects in the selective perfusion group had a deep embolic stroke on the side used for selective perfusion [75]. This high rate of an unusual neurologic injury is contrasted with a cohort of 57 neonates with high‐risk lesions, all undergoing selective cerebral perfusion from Texas Children’s Hospital. In that observational cohort, a 40% incidence of new injury was seen, with WMI being the most common, and none had evidence of unilateral embolic load, despite a high flow rate during selective perfusion (56 ± 10 mL/kg/min). In the Texas cohort, the duration of selective perfusion was not associated with poor neurocognitive outcome, but the duration of circulatory arrest was associated with significant worsening in the cognitive domain at 1‐year follow‐up [8]. Adding to the debate over whether to use hypothermic circulatory arrest or selective cerebral perfusion is the problem of choosing a flow rate when perfusing the circle of Willis directly. In the normal state of perfusion, the brain is protected from over‐ and under‐perfusion, specifically by the mechanism of pressure autoregulation. There is evidence that pressure autoregulation is entirely pressure‐driven and that cardiac output (or pump flow rates) is not relevant to cerebral perfusion as long as perfusion pressure is within the limits of autoregulation [76–78]. However, the ability of the cerebral vasculature to increase and decrease flow is at least conceptually dependent on a parallel vascular connection with the rest of the systemic circulation from which it can draw flow when vasodilating and to which it can push flow when vasoconstricting. With the great vessels snared and flow directed to the carotid artery, there is an unknown narrowing of the range of flow rates that yield pressures acceptable for cerebral perfusion. Inconceivably wide ranges of selective perfusion flow rates have been published, from 10 to 100 mL/kg/min [79, 80]. Experimental work in piglets has addressed this question. DeCampli, Myung and colleagues, and Sasaki and colleagues independently studied similarly aged infant piglets with selective perfusion techniques at different flow rates [81–83]. By recording arterial blood pressure at the limb ipsilateral to the cannulation site, it was possible to see the relationship between flow rates and resultant pressure directed to the cerebral vasculature (see Figure 14.9). Published reports of neonates studied during selective cerebral perfusion have recorded the right radial arterial blood pressure during right innominate cannulation, which allows for the same relationship to be described in humans (see Figure 14.9) [16, 80, 84–87]. Although the piglet data suggests a safe range of flow rates between 20 and 30 mL/kg/min, examination of the human data clearly shows that the piglet data cannot be translated in this manner. The human infant achieves a lower cerebral perfusion pressure for the same flow rates when compared with the piglet during selective perfusion. Further, the use of pH‐stat blood gas management in the human infant increases the required flow rate, whereas pH‐stat blood gas management in the piglet does not seem to change flow rate requirements during selective perfusion. Figure 14.9 The relationships between arterial blood pressure (ABP) and pump flow rates are shown for the piglet model of selective cerebral perfusion and the human infant for two blood gas management strategies. Table 14.1 Human vs. neonatal piglet models of selective cerebral perfusion The translation of piglet studies of cerebral perfusion is best understood after comparing the relative mass of the piglet and human brain, the relative body mass of the piglet and human infant, and cerebral blood flow expressed in standard units (mL/100 g/min), and in units relevant to perfusion (mL/kg/min). The effect of blood gas management is also shown. The human brain under pH‐stat management is predicted to require six times the pump flow rate of a piglet under α‐stat management. The effect of noncerebral tissue vasculature remains unknown. The translation of selective perfusion data from piglet to human would require accounting for differences in brain size (60 g piglet brain, 450 g human infant brain), brain development (the piglet is born ambulating with myelinated white matter, with different metabolic requirements and CBF), as well as the ratio of cerebral to collateral systemic circulation that occurs due to anatomic differences. Given the observed response to blood gas management changes shown in Figure 14.9, one can argue that the ratio of cerebral to noncerebral perfusion in the human during selective perfusion is much higher than the piglet model of selective perfusion. The basis of this argument is that CO2 tension changes in the blood affect cerebral vascular tone, but leave noncerebral vascular tone relatively unchanged. If the piglet had a large percentage of CBF to total blood flow during selective perfusion, the pressure would drop with pH‐stat management, as it does in human infants. CBFs during bypass have been measured in piglets and human infants under both alpha‐stat and pH‐stat blood gas management and are summarized in Table 14.1 to facilitate comparison of the human infant with the piglet model [33, 83, 88–92]. The piglet has a higher CBF/100 g of tissue, but pump flows are determined by body weight, not brain size. Therefore, the CBF rates/kg body weight are much smaller. These differences limit the applicability of piglet studies of selective perfusion to clinical practice. An alternative approach has been to rely on neuromonitoring of individual patients to determine selective perfusion flow rates. The TCD is used to determine flow velocities in the middle cerebral artery after cooling, but before selective perfusion. With initiation of selective perfusion, flows are gradually increased from zero until the flow velocities are equal to the preselective perfusion values [80]. This technique results in a wide interpatient variability in flow rates, with a mean flow rate of 56 ± 10 mL/kg/min. Of 57 high‐risk patients reported with this technique, 23 (40%) had a new MRI‐detected brain lesion after surgery. Most lesions were WMI, and the lesions were evenly divided between ipsilateral and contralateral hemispheres with respect to the cannulation site. Nonrandomized neurodevelopmental outcomes using the Bayley Scales of Infant Development at 12 months yielded cognitive domains on a par with population norms, and language and motor domains 0.8 and 0.9 standard deviations (SDs) lower than population norms [8]. If a man will begin with certainties, he shall end in doubts: but if he will be content to begin with doubts, he shall end in certainties. Francis Bacon, 1605 [93] Empiricism, inductive reasoning, and the scientific method have converted medicine from mysticism to an evidence‐based practice. The randomized controlled clinical trial is not new to medical science, but the modern ability to assimilate the vast catalog of medical data has been transformative [94]. The contemporary concept of evidence‐based medicine by meta‐analysis is a rigorous, methodical scoring of available evidence that provides a common platform for the standardization of practice [95]. Neuromonitoring has been reviewed with this matrix. In the case of all three neuromonitors presented in this chapter (electroencephalography, TCD ultrasonography, and reflectance NIRS), the final evidentiary scores were level III, i.e., no demonstrable benefit [44]. The antithesis of evidence‐based practice includes two categories of offense: nonconformity to practices shown to be beneficial and adherence to practices not shown to be beneficial. While no monitoring device has been shown by this evidentiary standard to be beneficial, the American Society of Anesthesiologists continues to recommend a standard, minimum acceptable intraoperative monitoring platform to include pulse oximetry, blood pressure, and electrocardiography [96]. This breach of evidence‐based practice is most pointed for the pulse oximeter, which is a mandatory intraoperative monitor despite failure of research to show any outcome benefit after randomization of nearly 23,000 subjects [97]. The universal refusal of practitioners to disbelieve the apparent benefit provided by pulse oximetry casts doubt on the ability of the randomized trial to demonstrate benefit for any monitoring modality [98]. For similar reasons, final conclusions in meta‐analyses of intraoperative neuromonitoring, including those described hereafter in this chapter, are commonly upgraded in tone from “not indicated” to “may be considered,” despite the inability to support this conclusion with data [44, 99]. The standard electroencephalogram (EEG) with 2–16 channels has been utilized in congenital heart surgery [100]. The EEG has a primary clinical purpose of detecting and diagnosing seizure activity. More recently, it has been used as a guide of anesthetic depth and to document electrocerebral silence before circulatory arrest [101]. EEG is affected by several factors, including anesthetic agents, temperature, and CPB. Impracticalities associated with intraoperative EEG include electrical signal interference, complexity of placement, and interpretation. Newer devices using processed EEG technology are more user‐friendly and have been extensively reviewed [102, 103]. Although the value of perioperative EEG monitoring has better rationale in recent years (see below), there is conflicting outcome data. For example, neonates after stage I repair of HLHS had normal perioperative EEG findings when postoperative MRI scans showed ischemic injuries [20]. The Bispectral (BIS™) Index Monitor (Covidien, Inc., Mansfield, MA, USA) is currently promoted to guide the depth of anesthesia. BIS sensors are applied to the forehead and temple, producing a frontotemporal montage, which connects to a processing unit. The device is easy to use, and the monitor requires no calibration. The proprietary algorithm of Covidien (originally Aspect Medical Systems) renders the BIS index from one‐channel processing of the EEG [104]. The BIS index ranges from 0 (isoelectric EEG) to 100 (awake), with mean awake values in the 90–100 range in adults, infants, and children [105]. While awareness is a concern with surgery requiring CPB in adults, there is a scarcity of data in the literature regarding this problem in children requiring heart surgery [106, 107]. The BIS monitor also reports a burst suppression ratio, which would, in theory, be useful to see the effect of cooling on suppression of electrical activity in the brain. However, the literature has only case reports describing this practice in pediatrics [108, 109]. In a cohort of children undergoing open heart surgery with an anesthetic tailored for “fast tracking,” BIS scores increased during rewarming, a period considered at risk for awareness under anesthesia [110]. However, in that study and a similar study of infants younger than 1 year, BIS did not correlate with biochemical surrogates of light anesthesia, including stress hormones. Figure 14.10 Intraoperative aEEG recording with repetitive ictal pattern indicating left‐sided seizure on the raw EEG trace (top row) and corresponding to the left compressed trace (third row) highlighted by the red arrow. (Source: Gunn et al. [113]. Reproduced with permission from Elsevier.) Andropoulos and colleagues reported a cohort of 68 neonates undergoing single ventricle (SV) and two‐ventricle (2V) repairs who underwent full 11‐lead neonatal EEG monitoring for 6 hours preoperatively and 72 hours postoperatively [111]. High‐flow bypass with neuromonitoring including NIRS with a treatment protocol for rSO2 <50% was utilized. Selective cerebral perfusion with transcranial Doppler‐guided flow rates were also employed, and DHCA was minimized. There were no preoperative EEG seizures identified. In the 72‐hour postoperative period, one patient experienced two brief EEG seizures for a total of 125 seconds; for an overall incidence of 1.5% (95% confidence interval 0.3–7.9%). This EEG seizure incidence is lower than most other cohorts (see below), and the discussion focused on targeted management of CBF and oxygenation and the fact that significant doses of benzodiazepines were administered intraoperatively and postoperatively in this population (median of about 2 mg/kg midazolam in the perioperative period), which could suppress seizures. This report emphasizes the importance of specifying anesthetic/sedative and bypass and neuromonitoring protocols when reporting EEG seizure incidence. Amplitude‐integrated EEG (aEEG) has been utilized in neonatal intensive care for two decades and has prognostic outcome value for term neonates with hypoxic–ischemic encephalopathy (HIE) and who undergo therapeutic hypothermia [112]. Four needle or adhesive electrodes plus a reference electrode are applied to yield a two‐channel EEG, from two parietal and two frontal locations. The raw EEG signal is smoothed, rectified, amplitude integrated, and displayed at the bedside. The aEEG signal is evaluated for background pattern, ictal discharges, burst suppression, low voltage, or an isoelectric EEG, allowing real‐time assessment and treatment. Reports of intraoperative use of aEEG for infant cardiac surgery are limited. Gunn and colleagues studied 39 neonates undergoing Norwood stage I palliation for HLHS with selective cerebral perfusion and reported intraoperative seizures in 9 (23%), predominately occurring at hypothermia of 20–24 °C [113] (Figure 14.10). Of note, NIRS was not utilized in this study nor was the CPB flow rate during selective cerebral perfusion specified (see above). Eighteen percent of this cohort experienced seizures in the early postoperative period. Seizures were not associated with later neurodevelopmental impairment at 2 years, but were associated with increased early mortality. Failure of the background aEEG pattern to return to normal by 48 hours was associated with increased early mortality and lower motor scores at 2 years. In other cohorts, postoperative abnormal aEEG patterns, consisting of seizures, abnormal background activity, and abnormal sleep–wake cycles, have been associated with new postoperative MRI brain injury after cardiac surgery in neonates and lower IQ but not motor scores at age 4 years [112, 114]. Pittet and colleagues reported 31 neonates with conventional 13‐ or 19‐lead video EEG monitoring before cardiac surgery, and 5 (16%) experienced EEG seizures, with no clinical correlate [115]. All neonates with preoperative seizures had significant brain injury on MRI, including acute stroke, watershed injury, intracerebral hemorrhage, and WMI. Of the 157 recorded seizures, 62% were in the same area as MRI brain injury. In a 2021 systematic review and meta‐analysis of DHCA and EEG monitoring in the perioperative period for pediatric cardiac surgery, Alkhatip and colleagues reviewed 19 studies with 1,267 patients [116]. The event rate of EEG seizures was 14.9%, and seizures were associated with acute neurological findings, MRI abnormalities, and lower IQ and PDI scores on longer term follow‐up. Longer DHCA times were associated with a higher incidence of EEG seizures. While intraoperative utilization of either conventional or aEEG monitors remains limited due to complexity and space constraints, postoperative EEG monitoring for high‐risk neonates and infants undergoing cardiac surgery is increasingly common, and the American Clinical Neurophysiological Society recommends continuous EEG monitoring in this patient group [117]. It seems intuitive to treat EEG seizures since they are clearly a manifestation of brain injury, and many centers are now doing this; whether seizure treatment and suppression will improve longer term neurodevelopmental outcomes needs to be further studied [118].
CHAPTER 14
Neurological Monitoring and Outcome
Introduction
Cerebrovascular physiology during cardiac surgery
Cooling and rewarming
pH‐stat vs. alpha‐stat blood gas management
Hemodilution and transfusion practices during CPB
Temperature management
Glucose management
Circulatory arrest
Selective cerebral perfusion
Selective cerebral perfusion and flow rates
Study subject
Brain weight (g)
Body weight (kg)
PaCO2 Mgt
CBF/brain wt
(mL/100 g/min)
CBF/body wt
(mL/kg/min)
Piglet
60
3
α‐stat
25
5
Piglet
60
3
pH‐stat
60
12
Human infant
450
3
α‐stat
12
18
Human infant,
450
3
pH‐stat
20
30
Neurological monitoring during congenital heart surgery
Electroencephalographic technologies
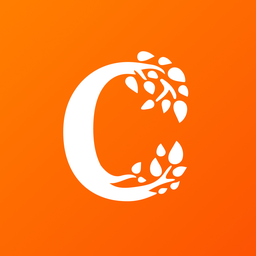
Full access? Get Clinical Tree
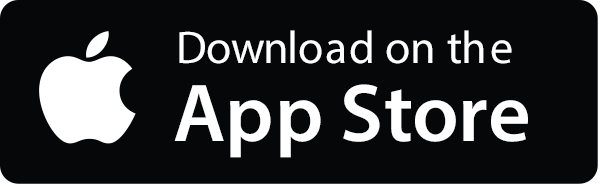
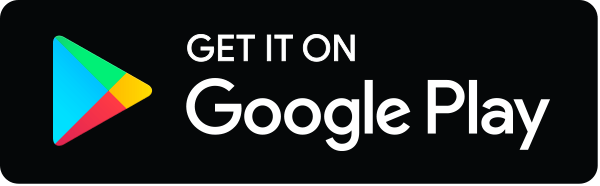