Gina Whitney1, Nicholas Houska1, Megan Albertz1, Brian Donahue2, and Suanne Daves3 1 Division of Pediatric Cardiac Anesthesiology, University of Colorado School of Medicine and Children’s Hospital Colorado, Aurora, CO, USA 2 Division of Pediatric Cardiac Anesthesiology, Vanderbilt University School of Medicine, Nashville, TN, USA 3 Department of Anesthesiology, University of Oklahoma College of Medicine, Oklahoma City, OK, USA The diagnosis and surgical management of congenital cardiac disease in the pediatric patient present a profound challenge to a developmentally immature physiology. Associated congenital syndromes, chronic abnormalities in hemodynamics, and immature developmental physiology of the young child all result in the potential for multisystem aberration. The exposure of a patient in this relatively tenuous state to the systemic challenges associated with cardiopulmonary bypass (CPB) results in both short‐ and long‐term challenges in the management of these children. CPB triggers complex cellular and humoral inflammatory responses designed to respond to noxious stimuli (Figure 12.1). Children are particularly prone to the effects of this response, due to the large relative surface area of bypass circuit, increased metabolic demand requiring higher flows, and developing organ systems particularly prone to injury [1]. These consequences of CPB predispose the pediatric patient to end‐organ injury and multisystem organ failure (MSOF). The management of this end‐organ dysfunction, which includes coagulopathy, pulmonary and myocardial dysfunction, renal insufficiency, and diffuse capillary leak, represents a major challenge in postoperative management. Activation of complement, the kinin–kallikrein–bradykinin system, and the coagulation system results in a systemic response that is both highly redundant and multisystemic (Figure 12.2). These events are triggered immediately upon contact of the patient’s blood with the foreign surfaces of the CPB circuit. Further activation occurs as a result of mechanical stress on blood components, hypotension, nonpulsatile perfusion, hypothermia, intestinal hypoperfusion, and systemic reperfusion following the release of the aortic cross‐clamp. Additionally, the administration of heparin and protamine during the course of cardiac surgery results in an increase in cellular and humoral processes that contributes to systemic inflammation and end‐organ injury [1]. Figure 12.1 Summary of the multiple systemic pathways involved in the physiologic response to cardiopulmonary bypass (CPB). Activation of the contact system by elements of the CPB circuit produces an array of physiologic humoral activations. Dilution of coagulation elements by crystalloid solutions decreases coagulability of blood and contributes to bleeding risk. Alterations in flow, such as ischemia–reperfusion injury, and nonpulsatile flow produce changes in endothelial function, such as expression of tissue factor and disordered endothelial‐dependent relaxation. Inflammatory cells, platelets, complement fragments, and cytokines mediate many of these pathways. Figure 12.2 Diagram showing the initiation of the inflammatory cascades produced by contact of blood with nonbiologic elements of the CPB circuit. Note the central role of kallikrein. Exposure of blood to the components of the CPB circuit results in rapid activation of complement via the alternate pathway and initiates inflammation. While this component of the complement system is designed to respond to microbial cell‐surface components and mediate the body’s defense against microorganisms, it may also be activated by exposure of blood to foreign surfaces, endotoxin, and kallikrein [2]. This activation results in conversion of C5 and C3 to C5a and C3a, respectively. C3a and C5a are potent anaphylatoxins that activate and degranulate neutrophils and mast cells and initiate platelet aggregation. C5a and C3a also act directly on endothelium, producing increased vascular permeability and smooth muscle contraction. C5a primes monocytes and macrophages to greatly increase the production of tumor necrosis factor alpha (TNF‐alpha) in the presence of endotoxin. This initial increase in TNF‐alpha may represent an early central event in the upregulation of the systemic inflammatory response [3]. In addition to the activation of the alternate complement pathway by contact with foreign surfaces, the classical complement pathway further contributes to the systemic inflammatory response when activated by heparin–protamine complexes. Their presence results in generation of C4a and further increases in C3a production, resulting in increasing vascular permeability. These processes (C5a and C3a activation, TNF‐alpha production) result in successive surges in humoral mediators of the inflammatory response: toxic oxygen metabolites, pro‐inflammatory cytokines (interleukin‐1 [IL‐1], TNF‐alpha, IL‐6, IL‐8, and lipopolysaccharide [LPS]), and anti‐inflammatory cytokines, which limit systemic inflammation (IL‐4, IL‐10). The TNF‐alpha levels increase dramatically following the initiation of CPB and peak at 2 hours and 18–24 hours postoperatively [4]. The levels of C5a and C3a are directly related to the duration of CPB, and infants exhibit a more dramatic increase than neonates [5]. Despite the known physiologic effects of these inflammatory mediators, attempts to correlate clinical outcomes with the degree of complement activation (using C3a and C5a plasma levels as markers) have led to inconsistent results [6, 7]. Kinins are polypeptides generated from kininogens following tissue injury or noxious stimulation. Their functions are incompletely understood, but are known to be involved in blood pressure regulation and mediation of inflammation through activation of G‐protein–coupled B1 and B2 receptors. Kinins are potent vasodilators, which also increase vascular permeability, smooth muscle contraction, and neutrophil chemotaxis. While B2 receptors are constitutively expressed in most tissues, B1 receptors are induced and functionally expressed in the presence of inflammatory cytokines, endotoxin, and following tissue injury. The generation of kinins depends upon the contact of blood with the negatively charged surfaces of the CPB circuit and subsequent activation of factor XII (FXII) to FXIIa. FXIIa results in the production of kallikrein from prekallikrein, which then results in further activation of FXII and the production of bradykinin from high‐molecular‐weight kininogen (HMWK). This positive feedback loop amplifies the production of bradykinin, a potent mediator of both acute and chronic inflammation. Bradykinin, in turn, results in further generation of inflammatory cytokines and the endothelial release of nitric oxide and tissue‐plasminogen activator. The response to CPB is also mediated by various cytokines, which facilitate communication between components of the immune and inflammatory systems. They are polypeptide hormones of low molecular weight (5–20 kDa) produced by macrophages, monocytes, lymphocytes, fibroblasts, and endothelium. They modulate humoral and cellular immunity in addition to the maturation and activation of immune cells. The plasma concentration of cytokines at baseline is in picomolar concentrations (10–12) and may increase up to 1,000‐fold in the setting of injury or infection. Data regarding the degree of cytokine release following CPB and the ratio of pro‐inflammatory versus anti‐inflammatory cytokines are often conflicting owing to inconsistencies in the source, technique, and the timing of sampling. It is clear, however, that identifiable waves of cytokine release characterize the inflammatory response to CPB and that important clinical and laboratory consequences of this response are observable in the perioperative period. Additionally, the functions of individual cytokines are highly redundant and pleiotropic, which presents a significant challenge to those looking to mitigate their downstream effects for clinical benefit. Normal endothelium is an important custodian of smooth muscle, producing vasoactive mediators such as nitric oxide and prostacyclin, which fine‐tune microcirculatory function. Injury to this critical monolayer decreases the production of these vasodilatory mediators and increases the release of vasoconstrictors endothelin and thromboxane A2 [8]. Endothelin probably mediates increased pulmonary vascular resistance, as higher plasma levels are found in patients who develop pulmonary hypertension. In a swine model of renal injury following CPB, an endothelin‐1 (ET‐1) receptor antagonist reversed many changes associated with endothelial dysfunction and renal injury [9]. The pulmonary endothelium is responsible for breakdown of several potent vasoconstrictors: angiotensin, catecholamines, and eicosanoids. Its injury therefore results in sustained increases in pulmonary vascular resistance following CPB. Endothelial dysfunction results in increases in capillary permeability and interstitial edema in addition to microcirculatory dysfunction. Efforts to improve pulmonary flow during CPB [10, 11], or supplement the NO pathway with L‐arginine [12, 13], may contribute to minimizing post‐CPB pulmonary pathology. Figure 12.3 Neutrophil adhesion, diapedesis, transendothelial migration and chemotaxis. ICAM, intercellular adhesion molecule. Neutrophil activation plays a pivotal role in ischemia–reperfusion injury and destruction of the extracellular endothelial cell matrix. CPB produces widespread activation of neutrophils and increased expression of adhesion molecules on neutrophils and endothelium. Activated neutrophils adhere to endothelium and migrate across interendothelial junctions to produce their effects in subendothelial tissues. Selectins, integrins, and immunoglobulins mediate neutrophil adhesion, migration, and chemotaxis (Figure 12.3). Notably, selectin expression increases substantially following CPB in pediatric patients and may be associated with clinical outcomes [14, 15]. Additionally, IL‐1 and TNF‐alpha result in increased ELAM‐1 (endothelial leukocyte adhesion molecule‐1) expression on endothelium, facilitating further the binding of neutrophils to the endothelium. Additionally, IL‐8 promotes interaction of activated neutrophils with endothelial ICAM‐1 receptors and results in further neutrophil migration to the extravascular space. CPB decreases gastric mucosal pH and intestinal perfusion and increases intestinal permeability. Endothelial dysfunction decreases the release of nitric oxide and prostacyclin, thus increasing the vascular resistance in splanchnic beds. The resulting intestinal hypoperfusion allows translocation of predominantly Gram‐negative bacteria. Endotoxin, the LPS component of the bacteria outer cell membrane, binds macrophages and monocytes and triggers further release of inflammatory mediators. Endotoxin is present in the circulation of 96% of children following CPB [16]. Endotoxin is a recognized prologue of inflammation in the setting of enteric pathology. Circulating endotoxin and inflammatory mediators (IL‐1, IL‐1beta, and IL‐8) correlate with the severity of necrotizing enterocolitis (NEC) in newborns [17]. Children with greater endotoxin burden have higher serum levels of IL‐6, more hemodynamic instability, and greater mortality [16]. Data suggest that milrinone may improve GI blood flow and thereby reduce endotoxemia and IL‐6 levels in adults undergoing CPB [18]. Additionally, hypothermia during CPB has been associated with increased levels of endotoxin [19]. Hemostasis is extensively altered during cardiac surgery. CPB produces widespread perturbations in platelet function, thrombin activation, endothelial function, and inflammatory cells. The familiar “coagulation cascade” (Figure 12.4) has served for years as an important educational paradigm in many respects. It has led to the development of our common coagulation tests, which have been useful in identifying deficiencies in the coagulation pathways. It has also been useful in identifying coagulation inhibitors. Yet, while abundantly referenced formally and discussed informally, the traditional cascade model does not explain some essential observations. For example, patients with FXII or prekallikrein deficiency typically do not have bleeding problems, a conclusion unlikely to be drawn by tracing the pathways of the cascade. Likewise, patients with FVIII or FIX deficiencies (hemophilias A and B) have severe coagulopathies, also unexplained directly by the cascade. The cascade model does not account for the roles of cells and tissues where the coagulation reactions occur. Consequently, pedagogy in coagulation has shifted toward the cell‐based model of coagulation, originally proposed by Hoffman and Monroe in 2001 [20] (Figure 12.5A and B). It is useful to carefully step through the cell‐based model, because doing so will explain the abovementioned limitations in the traditional cascade model. In this revised model, coagulation proceeds by the processes of initiation, amplification, and propagation [20–22]. In the initiation phase, elements of the intrinsic pathway (FXII, FXI, prekallikrein, HMWK), which are important for the generation of kinins as mentioned above and for initiating coagulation in a laboratory glass vessel, are not essential for the initiation of coagulation in vivo. Rather, the initiation phase begins when FVIIa contacts tissue factor (TF) at the site of vascular injury. The resulting TF–FVIIa complex activates FX and FIX. The resulting FXa is sufficient to generate a small amount of thrombin (FIIa), localized to the site of injury, since FIIa diffusing away from the site is significantly dampened by antithrombin (AT), and the FVIIa–TF–FX complex is rapidly inhibited by tissue factor pathway inhibitor (TFPI). Figure 12.4 The familiar coagulation cascade model showing intrinsic and extrinsic pathways. Rectangles denote enzymes or their corresponding zymogens, and ovals represent cofactors. As mentioned in the text, this model has provided much utility in the past, but fails to account for some simple clinical observations. Examples include failure to fully explain why contact factor deficiencies are not associated with significant bleeding and why factor VIII and IX deficiencies are associated with severe bleeding. In the amplification phase, the thrombin that is present activates FV and FVIII, important cofactors for FXa and FIXa, which form complexes on platelet surfaces to increase further generation of thrombin. Platelet activation results in a proliferative increase in phospholipid surface rich in phosphatidylserine, essential for these enzymatic complexes to bind and function, and produces secretion of additional coagulation factors (FV, FVIII, von Willebrand factor [vWF]) from platelet granules [23]. Thrombin also provides an important positive feedback loop by activating FXI, which also generates additional FIXa. Once these elements are in place (activated platelets, activated cofactors FVa and FVIIIa, thrombin, and FXa and FIXa), the propagation phase is marked by sustained thrombin generation provided by the prothrombinase complex (FXa–FVa). The success of this phase depends mostly on activity of the tenase complex (FVIIIa–FIXa), which generates continued amounts of FXa. The endpoint of coagulation is this local, sustained generation of thrombin, which catalyzes conversion of fibrinogen into fibrin monomer, spontaneously polymerizing into fibrin strands to form a stable clot. Thrombin also activates FXIII, which cross‐links and stabilizes fibrin clot, increases neutrophil adherence to endothelium, and stimulates neutrophil release of chemotactic and inflammatory cytokines. Coagulation is normally confined to the region of injury by several mechanisms. Inactivated platelets do not express significant levels of phosphatidylserine on the plasma surface of their membranes and, therefore, do not support coagulation factor activity very well. Upon activation, the platelet outer membrane expresses phosphatidylserine and serves as a platform for the formation of coagulation complexes. Normal endothelium does not express TF, nor does it contain appreciable amounts of exposed phosphatidylserine and, therefore, does not support coagulation. Therefore, any FVIIIa–FIXa or FVa–FXa that diffuse away from the site of injury will not generate thrombin. AT and heparin cofactor II rapidly attenuate any stray FIIa. Thrombin also forms a complex with thrombomodulin on endothelial surfaces, activating protein C, an important control element that cleaves FVa and FVIIIa into inactive fragments. Figure 12.5 (A) Widespread platelet activation is triggered in the setting of cardiopulmonary bypass (CPB) by the activity of c5a, thrombin, and the secretion of platelet activating factor (PAF) by endothelium. Platelet activation results in a dramatic change in platelet morphology, exocytosis of dense granules and alpha granules, and aggregation. Aggregation is mediated by von Willebrand factor (vWF) and glycoprotein IIb/IIIa receptors that bind fibrinogen. The phospholipid surface of the platelet is the site of assembly of enzyme complexes essential to clot formation. (B) The revised model of coagulation, the cell‐based model. Essential to understanding this pathway is the assembly of enzyme complexes on phospholipid surfaces with the requirement for calcium ions. The pathway consists of three overlapping phases: initiation, amplification, and propagation. In the initiation phase, exposure of blood to tissue factor (TF) produces a small amount of activated factor X (FXa). This, with FV results in the formation of a small amount of local thrombin (FIIa). In the amplification phase, this amount of thrombin is insufficient to produce significant fibrin clot, but it activates FV and FVIII, greatly increasing their activity, and activates FXI, which provides a positive feedback loop by activating FIX. Platelets are activated by contact with the site of injury and provide a massively greater phospholipid surface for the assembly of the complexes in the propagation phase. Here, sustained thrombin generation is maintained by continued generation of the FVIIIa/FIXa complex and the prothrombinase complex (FVa/FXa). CPB produces profound alterations in coagulation factor levels and activities, which are quantitatively different in children when compared to adults [24–27]. Because of the large relative pump‐prime volume, hemodilution is a much greater cause of decreased factor levels after CPB in children [27]. Dilution and consumption of coagulation factors is the major cause of post‐CPB coagulopathy in children [28]. Despite high levels of heparin during CPB, thrombin is generated [25, 29]. It is tempting to assign thrombin generation during CPB to activation of the contact pathway, since blood is exposed to the nonbiologic surfaces of the CPB system. However, patients with contact factor deficiencies (FXII, prekallikrein, FXI) still manifest thrombin generation during CPB [30, 31], indicating that the contact pathway is not driving coagulation during CPB. Rather, exposure of blood to TF is likely the cause. Inflammatory cytokines produce TF expression on endothelium and monocytes, and contact of blood with the nonendothelialized tissues in the mediastinal cavity and elsewhere produces thrombin activation [32]. Thrombin then perpetuates both inflammatory and thrombotic processes. This prothrombotic state may persist for several hours postoperatively, even in the setting of clinical bleeding. In infants, TF expression may extend into the postoperative period [32]. Platelets are activated during CPB and extrude their contents [1, 24]. Glycoprotein receptors become expressed on the platelet surface. Released factors include platelet factor‐4, beta‐thromboglobulin, and vWF from alpha granules, while the platelet surface expresses adhesion molecules such as P‐selectin. Platelet activation and adhesion to endothelium results in additional expression of transmembrane receptors that mediate binding and transmigration of activated neutrophils. This results in additional recruitment of neutrophils to the extravascular space. Furthermore, platelets of young infants are generally less reactive than those of older children and adults, and decreased platelet activation, as measured by changes in adhesive receptor density, has been observed following CPB in infants [33]. Recent attention has been paid to acquired von Willebrand disease (aVWD), represented by loss of high‐molecular‐weight vWF multimers, in children with congenital heart defects. Reportedly, aVWD is common in this population, both before and after surgery [34–36], but interestingly did not seem to correlate with clinical evidence of bleeding, possibly since endothelial secretion of vWF occurs during surgery due to the stress response [35–37], thus attenuating the effect of a preexisting aVWD. The frequency of aVWD among pediatric patients on other means of extracorporeal circulation, such as extracorporeal membrane oxygenation (ECMO) or ventricular assist device (VAD), has been reported at 100% [38], potentially implicating the role of shear stress on blood components as a possible contributor to loss of the high‐molecular‐weight multimers of vWF. See Chapters 11 and 16 for further discussion of bleeding and coagulation. Practitioners have sought to develop effective means of mitigating the systemic response following CPB in children. Practices include perioperative corticosteroids, modified ultrafiltration (MUF), protease inhibitors, and biocoating of CPB circuitry. Although many such strategies achieve reduction in the levels of various inflammatory markers, studies demonstrating improvement in relevant clinical outcomes show more modest results. Two methods commonly in use include perioperative glucocorticoids and MUF. It is clear that effective modulation of the systemic inflammatory response to CPB in clinically meaningful ways remains elusive. Perioperative corticosteroids are often intended to reduce the systemic inflammatory response and its deleterious effects. Corticosteroids act through nuclear transcription factors, whose binding to promoter regions decreases expression of pro‐inflammatory mediators. While studies have demonstrated reductions in inflammatory mediator expression, there is little consensus on the clinical benefits of steroid administration in this setting. Furthermore, there is tremendous heterogeneity among the patient management regimens, and sample sizes are often small. These issues complicate the efforts of systemic reviews and limit their utility. Thus, a comprehensive review of steroids to minimize the impact of CPB is beyond the scope of any one chapter. With this in mind, however, it is possible to summarize some common themes in our current understanding. In 2012, Pasquali et al. conducted an analysis of data from the Pediatric Health Information Systems (PHIS) and Society of Thoracic Surgeons Congenital Heart Surgery (STS‐CHS) Databases [39]. They reported results from 30 pediatric cardiac centers across the United States from 2004 to 2008, comparing outcomes of neonates receiving methylprednisolone on the day prior to or on the day of surgery. The study population included 3,180 neonates from 25 centers. They found no difference in mortality or length of hospital or intensive care unit (ICU) stay between the methylprednisolone and control groups. More recently, Bronicki and colleagues [40] published their findings from a meta‐analysis of randomized prospective trials involving steroid treatment to mitigate the impact of inflammation in pediatric CPB. Their findings showed a significant heterogeneity between trials, necessitating the use of a random‐effect model. Nonetheless, the authors observe a significant improvement on short‐term fluid balance associated with steroid treatment, but no measurable impact on infection or mortality. Likewise, a recent Cochrane review [41] reported findings from a meta‐analysis of current pediatric steroid trials, showing that steroid therapy may decrease the duration of mechanical ventilation, but did not show a measurable impact on mortality. Such recent data regarding the lack of clinical effectiveness of perioperative steroids is leading many centers to reconsider their routine use. Hypothermia, hemodilution, and the profound inflammatory response to CPB result in widespread capillary leak and increase in total body water. Dilution of plasma proteins, particularly in the smallest patients, results in further increase in interstitial fluid. Management of total body fluid overload can be especially challenging in the postoperative period due to concomitant myocardial dysfunction and renal immaturity often seen in the pediatric patient. MUF removes water, low‐molecular‐weight solutes, and inflammatory mediators via filtration immediately following CPB. It also allows return of the contents of the CPB circuit to the patient in a concentrated form [42]. MUF has been shown in an animal model to attenuate pulmonary‐derived inflammatory markers (based on reductions in IL‐6 and IL‐8 concentrations obtained by bronchoalveolar lavage) seen following bypass. In this model, MUF reduced pulmonary vascular resistance [43]. MUF has been shown to be effective in reducing weight gain during CPB, decreasing levels of activated complement C3a and C5a, and reducing concentrations of pro‐inflammatory cytokines [44]. While some studies have shown little appreciable difference in the levels of inflammatory mediators following MUF, others have shown increase in plasma concentrations of IL‐6, IL‐8, and neutrophil elastase [45]. Clinically, MUF reduces total body water, decreases transfusion requirement, reduces postoperative coagulopathy, and decreases inflammatory marker levels after CPB [46]. A 2011 survey of international pediatric perfusion practice found that 71% of centers reported using some form of MUF [47]. While the clinical outcome differences associated with MUF are not dramatic, the beneficial effects of MUF may be more easily demonstrated in neonatal patients requiring a longer duration of CPB [48]. Protease inhibitors are a class of drugs whose primary role is inhibition of various signaling pathways. Aprotinin, originally marketed as a kallikrein and plasmin inhibitor, decreases transfusion requirements following CPB, but also has substantial anti‐inflammatory effects [49, 50]. Two randomized trials have shown decreased hospital stay and cost associated with aprotinin [51, 52]. Another blinded, controlled, randomized, prospective trial in 60 children weighing under 10 kg reported decreased ventilator time [53]. Both aprotinin and tranexamic acid (not a protease inhibitor) reduce expression of pro‐inflammatory genes and increase expression of anti‐inflammatory proteins [54]. Since 2006, aprotinin use has severely declined, and aprotinin is now unavailable in many countries, including the United States. The departure of aprotinin has been driven mostly by epidemiologic evidence for renal failure in adults [55–58]. Whether the same risk transfers to the pediatric population is unclear: Three retrospective studies did not identify aprotinin as an independent risk factor for perioperative renal failure in children [59–61]. However, another retrospective pediatric study reported an association between aprotinin and perioperative renal failure when compared with aminocaproic acid, after controlling for confounding variables [62]. Efforts to improve CPB circuit biocompatibility are often anticipated to decrease activation of cellular and humoral inflammation and attenuate end‐organ injury associated with CPB. Although the initial rationale for heparin coating of CPB circuitry was related to the anti‐thrombotic effects of heparin, the use of heparin‐coated CPB circuit components has reduced complement activation, cytokine production, and improved pulmonary function [63–65]. Heparin‐coated circuits also appear to adsorb lipoproteins, which may create a circuit surface that approximates that of cell membranes [66]. A recent small randomized study of perfusion circuits coated with a novel ternary polymer (SEC‐1) showed significantly decreased inflammatory markers and improved preservation of multiple coagulation elements [67]. Though definitive data on clinical outcomes in children regarding heparin‐bonded circuits are lacking, adult data suggest that biocompatible circuits are associated with reduction in RBC transfusion, re‐sternotomy, duration of mechanical ventilation and ICU length of stay, and inflammatory response following CPB [68, 69]. The clinical impact of biocompatible perfusion circuitry in children warrants further study, particularly in high‐risk patients. These major physiologic disturbances of the inflammatory and coagulation pathways produce significant morbidity in the form of hemorrhagic and thrombotic complications. Perioperative risk of bleeding and thrombosis increases in both severity and frequency with decreasing age of the child [26, 70–73]. Infants under 1 year of age experience average blood losses within the first 24 hours after surgery of about 40 cc/kg, which is about half of the patient’s blood volume [73]. Perioperative thrombosis is also associated with significant morbidity, sometimes severe [74–78]. Venous thrombosis in children is a potentially devastating complication of congenital heart surgery and is reported to occur in as many as 16% of cases [79–81]. Central venous thrombosis and massive thrombotic disease in multiple organs occur with highest frequency in the youngest children and can yield catastrophic sequelae [33, 82, 83]. It is important to understand that, like many other organ systems, the hemostatic system of young infants differs significantly from that of older children and adults [21, 84, 85], giving rise to a concept known as “developmental hemostasis” (Figure 12.6). Healthy infants exhibit levels of the contact factors (FXII and FXI, HMWK, prekallikrein) that are 40–50% of adult levels at birth, and approach adult levels by 6 months of age. This profile is also followed by the vitamin K‐dependent factors (FII, FVII, FIX, and FX) [84, 86–88]. FV, FVIII, and vWF reach adult levels more rapidly, and, as is the case for vWF, can exceed adult levels. Coagulation inhibitors (AT‐III, C1 inhibitor, heparin cofactor II, protein C, and protein S) again follow the pattern of the contact and vitamin K‐dependent factors [84]. Fibrinogen and plasminogen, although present at nearly adult levels in the term newborn, exist in forms that differ in their post‐translational modification [89, 90]. Accordingly, laboratory reference ranges need to be based on age‐matched samples from normal children. Congenital heart disease (CHD) imposes additional modifications on pediatric hemostasis (Table 12.1). In cyanotic children, polycythemia further dilutes coagulation factors. Decreased levels of FV, FVII, and FVIII and increased fibrinolysis, thrombocytopenia, and abnormal platelet function are frequently present and correlate with the degree of cyanosis [91]. Thrombocytopenia resulting from decreased platelet survival and fibrinogen dysfunction complicate the hemostatic picture and contribute to increased risk for bleeding [92, 93]. Children with CHD have increased incidence of heritable coagulopathies [94, 95] and combined deficiencies or partial deficiencies of FVIII, FXI, and FXII [96]. As mentioned above, loss of high‐molecular‐weight vWF multimers, representing a form of aVWD, is often observed in children with cardiac defects [97]. Table 12.1 Effects of cyanosis on coagulation physiology Hemostatic anomalies of cyanosis are widespread and complex, and involve multiple elements of the coagulation and fibrinolytic systems [92, 93]. Numerous studies have identified clinical risk factors for hemostatic complications after pediatric cardiac surgery. In their classic study of over 500 pediatric patients, Williams et al. found that postoperative bleeding is associated with higher hematocrit, complex surgery, lower platelet count, and duration of circulatory arrest, but younger age was the most significant contributor [98]. Since then, other multivariate analyses have revealed that hypothermia, surgical complexity, high preoperative hematocrit, low platelet count, and decreased platelet function were highly correlated with blood loss and transfusion [70, 73, 98–101]. Some evidence suggests postoperative bleeding risk may vary among different ethnic groups [102]. Box 12.1 summarizes coagulation system changes in pediatric cardiac surgery. Figure 12.6 Developmental hemostasis. Factor levels in infants and younger children are generally lower than those of adults and evolve with age. Laboratory factor measurements and assays of coagulation need to be standardized for appropriate age groups. (Source: Data from Attard et al. [84].) Transfusion alone appears to exacerbate the systemic inflammation of cardiac surgery. Transfusion following cardiac surgery is associated with various markers of inflammation [103], and with clinical outcomes such as longer ventilator times [104–106]. Univariate analyses show that red cell transfusion is associated with nearly every complication of congenital heart surgery, and transfusion is strongly associated with infectious complications, after controlling for confounding variables in a propensity analysis [107]. The authors have argued transfusion‐related immunologic responses alone are good reasons to optimize transfusion management [105, 106, 108]. The rational approach to a bleeding pediatric cardiac surgery patient represents a significant clinical challenge for which the literature often provides limited prospective data. In a study with 75 children, platelet transfusion was most effective for post‐CPB bleeding in patients under 8 kg. Continued bleeding was improved with cryoprecipitate but was made worse by plasma in this study [70]. Plasma is often ineffective in children probably because higher doses are required than the usual 10–15 mL/kg [109, 110]. Plasma added to the bypass prime may be beneficial, but the effect seems confined to the smallest patients [111, 112]. Fibrinogen concentrate has been proposed as an alternative for cryoprecipitate, but efficacy appears to be best for the adult cardiac surgery population [113–117], as data in children do not currently support its use [118]. While transfusion is frequently required in children in order to maintain adequate oxygen delivery, efforts should be made to reduce the volume of transfusion required. In a recent systematic review [119] of 299 studies of transfusion optimization from 22 countries, the authors concluded that the following efforts have merit in managing perioperative bleeding: antifibrinolytic drugs, objective transfusion guidelines, point‐of‐care testing, MUF, and circuit optimization. A large amount of heterogeneity regarding end point definition, patient population, and management schema and frequently small trial sizes made definitive conclusions difficult. Still unclear is the issue of liberal versus restrictive transfusion thresholds. Although the literature on transfusion thresholds in adults is rapidly expanding, similar studies in children with CHD are fewer and less conclusive. Cholette [120] provides a recent summary of the pediatric cardiac surgery literature on restrictive versus liberal transfusion thresholds, summarizing the four existing studies [121–124] at the time. Despite concerns regarding exclusion criteria and small study size, the authors concluded that a more restrictive transfusion regimen (target hemoglobin 7 g/dL for biventricular repairs, 9 g/dL for cavopulmonary surgeries) may be well tolerated or even advantageous in these populations. They issue caution, however, that these results need to be validated and reproduced in larger trials and by other investigators before practice guidelines can be issued. Objective, laboratory‐guided transfusion algorithms have shown significant benefit in adult cardiac surgery [125], but it is difficult to extrapolate these conclusions to children, since adult and pediatric cardiac surgery are qualitatively different [126, 127]. Furthermore, not all reports of transfusion algorithms in children have shown positive results [128]. To be clinically useful, laboratory‐guided protocols must rely on some reproducible, widely accessible metric. Routine coagulation tests, thromboelastography (TEG), and platelet count can predict postoperative bleeding in children after CPB [129, 130], which assists clinicians somewhat in identifying patients at risk. The use of TEG to specifically guide transfusion practice in pediatric cardiac surgery has been reported, with generally favorable results [28, 117, 131, 132]. A 2‐year retrospective study of an objective transfusion algorithm in pediatric cardiac surgery has shown a significant savings of red cells, cryoprecipitate, and plasma and a 75% reduction in mortality following implementation of the algorithm [133]. In a recent review, the authors summarize a very broad literature, which includes the use of factor concentrates, but continue to emphasize the recurring observations that algorithms need to be institution‐specific and that a transfusion‐guided algorithm is superior to non‐algorithm‐guided transfusion [134]. Thrombin generation during CPB, and kinin activity from kallikrein activation, produce plasmin and thus activation of the fibrinolytic pathway. Accordingly, antifibrinolytic drugs (aprotinin, aminocaproic acid, and tranexamic acid) have long been useful adjuvants for limiting blood loss due to cardiac surgery. In general terms, their efficacy in children has been less consistent than in adults. Unlike adults, most children do not exhibit profound fibrinolytic activity after CPB [33, 72], and consequently, efforts to reduce bleeding by the use of antifibrinolytic drugs are usually less effective in children than in adults [52, 135–139]. Some evidence in children suggests that fibrinolysis alone does not correlate with blood loss or transfusion in children [72]. The literature on antifibrinolytics for pediatric cardiac surgery is very broad, with much heterogeneity in end point definition and treatment protocols; therefore, the interested reader is referred to an excellent recent review [140]. When it was still available, aprotinin has been used in pediatric cardiac surgery, despite lack of the Food and Drug Administration (FDA) approval for pediatric use. Endpoints of blood losses and transfusion appear not as striking as those for adults, and a wide range of dosing schemes has been reported. Many prospective pediatric trials report hemostatic benefits with aprotinin [141–143], but most show equivocal results [52, 135–138, 144–146]. One of these studies [146] was terminated early by the FDA due to growing safety concerns in adults [55–58]. Nevertheless, beneficial effects of aprotinin in children extend beyond hemostasis. As mentioned previously, decreased hospital stay, shorter ventilator time, and decreased cost have been associated with aprotinin in children [51–53]. These improvements are likely a result of the anti‐inflammatory effects of aprotinin, producing improved organ preservation and suppressed response to extracorporeal circulation. The lysine analogs (aminocaproic acid and tranexamic acid) have been the only antifibrinolytics in clinical use since 2006. The lysine analogs probably do have efficacy in children, but less than that of aprotinin [147–149]. As with aprotinin, the efficacy of lysine analogs in children has been inconsistently reported, and differs between subgroups. Pediatric use of aminocaproic acid has been reported, but many of these studies were performed in the 1960s and 1970s, when surgical, anesthetic, and perfusion techniques were quite different than they are today [150, 151]. Williams et al. compared aminocaproic acid versus placebo control in a nonrandomized study involving 140 children and demonstrated nonsignificant decreases in blood losses and transfusion requirements [152]. Tranexamic acid has shown efficacy in children undergoing redo cardiac procedures [139], both in cyanotic and noncyanotic patients [153]. An earlier study of pediatric use of tranexamic acid was only able to demonstrate a reduction in postoperative blood loss in a subset of cyanotic children [154]. In a nonblinded trial, both aminocaproic acid and tranexamic acid were found to be equally beneficial in 150 cyanotic children [155]. The overall conclusion regarding the currently available antifibrinolytics (lysine analogs) is that these agents are safe and effective in the pediatric population [140]. As mentioned previously, MUF concentrates hemostatic factors and removes inflammatory mediators, thus reducing postoperative coagulopathy [156]. MUF appears to be most effective at improving hemostasis for the smallest infants [48, 157, 158]. Bando et al. compared MUF after CPB against conventional ultrafiltration and observed a 2.5‐fold decreased risk of red cell transfusion and 6‐fold decreased risk of coagulation factor transfusion with MUF [48]. Other investigators have shown similar improvements in post‐CPB coagulopathy or transfusion requirements with MUF [159–163]. Additional benefits include improved hemodynamics and cardiac performance [159, 160, 163–165], decreased ventilatory support [48, 163], and shortened hospital stay [44, 162, 163] (Table 12.2). These effects have not been reported in all studies [157, 166]. A meta‐analysis of eight MUF studies showed improved hematocrit and hemodynamics compared with standard ultrafiltration, but later end points were equivocal [167]. Table 12.2 Reported benefits of modified ultrafiltration Modified ultrafiltration after CPB improves not only hemostasis and transfusion, but has benefit in other organ systems, possibly due to decreased levels of inflammatory mediators, decreased capillary leak, and improved myocardial performance [156]. Investigators have reported successful use of alterations in perfusion techniques, some of which avoid transfusion altogether, even in very small infants. Minimizing the volume of the pump prime [168–171], or use of a miniaturized bypass circuit [171, 172], has shown efficacy for reducing transfusion in small children. Alterations in pump composition such as a remote pump head resulted in no transfusion for 122 of 178 infants under 5 kg [173]. Use of washed red cells [174], fresh whole blood [71, 170, 175], heparin‐coated circuits [176], use of an autologous blood prime [177], and anticoagulation management based on heparin concentration rather than ACT [178] have all reported efficacy in the pediatric population. However, practice guidelines have yet to be issued for most of these techniques often due to small study size, heterogeneity of end point definition and patient management protocol, possibility of publication bias, and lack of reproducibility of the results. Recombinant activated factor VII (rFVIIa) has been used with increasing frequency as a rescue hemostatic agent in cardiac surgery for about the past two decades. Case reports of its use in pediatric cardiac surgery abound [179–183]. Serious concern exists regarding perioperative thrombosis, but the anecdotal nature of case reports and small case series makes it difficult to evaluate thrombotic risk associated with this agent, since it is typically administered to patients in dire need, who are likely to be at increased risk for thrombosis. To deal with this issue of confounding, a recent propensity analysis of 143 subjects receiving rFVIIa matched 1 : 2 with controls was reported by Downey and colleagues [184], who observed significant morbidity associated with the use of rFVIIa, including an independent risk for thrombosis. The rational use of rFVIIa in pediatric cardiac surgery has been reviewed [185, 186]; the authors emphasize the need for randomized trials to clarify efficacy, subgroup analysis of population at risk, and the realistic assessment of thrombotic complications. Furthermore, a Task Force has been formed by the Congenital Cardiac Anesthesia Society, assigned to monitor the literature and issue recommendations as data become available [186]. Low antithrombin‐3 (AT3) levels may place very young children at increased risk for bleeding, since such deficiency decreases the efficacy of heparin and therefore results in greater coagulation activation during CPB, with corresponding increase in coagulation factor consumption. Recent investigations have identified low AT3 as an independent risk factor for transfusion following cardiac surgery [29, 187], and a prospective trial of recombinant AT3 has reported efficacy specifically for small infants when this deficiency can be identified preoperatively [188]. Supplemental AT3 has also shown efficacy in the setting of ECMO [189]. Desmopressin has minimal hemostatic effects in adult cardiac surgery, and its specific impact in the pediatric population is probably also modest at best [190–193]. The use of other factor concentrates (fibrinogen concentrate, prothrombin complex concentrate) in pediatric cardiac surgery has been recently reviewed [118, 194]. These agents, initially proposed as rescue treatments for refractory hemorrhage, are now appearing in transfusion algorithms as standard treatments. The authors emphasize the need for high‐quality studies of safety and efficacy, with standardized definitions of outcomes, prior to issuing any evidence‐based recom‐mendations. Thrombosis is frustratingly common in children with CHD since it is associated with significant morbidity [74–78]. In a series of single ventricle patients, systemic‐to‐pulmonary shunt occlusion accounted for 9% mortality during a 6‐year follow‐up [195]. Other authors report a 14% post‐discharge mortality in a similar series, 81% of which was sudden and unexpected. Aspirin did not alter risk in this population [196]. In long‐term studies, thrombosis has been identified as the first [197] or second [198] most common cause of late mortality in Fontan patients. Echocardiographic evidence of thrombus exists in as many as a third of asymptomatic children following the Fontan operation [74], with venous thromboembolism and stroke occurring in 19% of these patients [78]. The vascular access necessary for catheterization procedures is associated with a high rate of thrombosis [78], and post‐thrombotic syndrome frequently complicates the clinical course [199]. Furthermore, reported incidence rates of thrombosis vary widely between institutions [74, 200–203], complicating comparisons between studies and interpretations of their results. Thrombosis has some identifiable risk factors. Petaja et al. describe thrombotic events heralded by hypofibrinolysis in a small series of 10 neonates undergoing cardiac operations [33]. In this study, central venous thrombosis was associated with increased PAI‐1, AT3, and protein C levels. In another case series of children with thrombosis and CHD, both acquired and inherited risk factors were found to be significant [200]. Some of these factors included multiple cardiac catheterization procedures, presence of a central venous line, pulmonary stenosis, aortic coarctation, and systemic infection. However, this study identified only 59 subjects with thrombosis and CHD, and an analysis of the interaction between environmental and genetic variables was not performed. Steen and colleagues recently confirmed the strong contribution of a central venous line to thrombosis risk in their cohort of 851 critically ill children [204]. Other risk factors identified in their cohort included longer ventilator times and ICU stay, low cardiac output syndrome, sepsis, catheter‐associated blood stream infection, and cardiac catheterization. Interestingly, CPB was observed to have a protective role in thrombosis in this population. Ali and colleagues recently reported thrombosis outcomes from their cohort of 369 pediatric subjects, 18% of whom experienced thrombosis [205]. Thrombosis in their population was associated with cyanosis, neonatal surgery, preoperative antiplatelet or anticoagulant therapy, and post‐CPB transfusion. Transfusion was also identified as a thrombotic risk factor in a previous study of 138 children [206]. In other reports, markers of inflammation such as elevated free heme [207] or C‐reactive protein levels [75] may also predict risk. Recent evidence is emerging that red cells probably contribute to coagulation activity [208], and some studies report that red cell transfusion increases thrombosis risk in pediatric surgery [208–212]. The role of genetic thrombophilias, such as FV Leiden (FVL), as a risk factor in this population has been evaluated, but the findings are difficult to interpret [81, 83]. Petaja’s group reported central venous thrombosis in 20 of 1,591 pediatric patients undergoing CPB [83]. Of these, 12 were tested for activated protein C resistance, and 2 were positive. Ong et al. [81] screened a group of 200 consecutive pediatric cardiac surgery patients for FVL, but found the mutation not to be significantly associated with thrombotic risk. Protein C sensitivity, the laboratory coagulation phenotype which is reduced in the presence of the FVL mutation, has been associated with the degree of cyanosis in children with CHD, independent of FVIII levels and the presence of FVL [213], suggesting that cyanotic children are at decreased risk for thrombosis. Box 12.2 summarizes risk factors for thrombosis.
CHAPTER 12
Multiorgan Effects of Congenital Cardiac Surgery
Introduction
The systemic response to congenital cardiac surgery
Systems mediating the systemic inflammatory response to CPB
Complement pathway activation
Activation of the kinin system
Activation of inflammation
Endothelial injury
Neutrophil activation and sequestration
Endotoxemia
Activation of the coagulation system
Old and new models of coagulation
CPB and the coagulation system
Mitigating the deleterious effects of CPB
Corticosteroids
Modified ultrafiltration
Protease inhibitors
Biocompatible CPB circuits
Clinical effects of congenital heart surgery on hemostasis and thrombosis
Unique aspects of pediatric coagulation
Hemostasis in congenital heart disease
Thrombocytopenia
Decreased platelet survival
Acquired von Willebrand disease
Fibrinogen dysfunction
Low‐grade consumptive coagulopathy
Decreased coagulation factor levels
Accelerated fibrinolysis
Risk factors for hemorrhagic complications in pediatric cardiac surgery
Transfusion, inflammation, and outcomes
Transfusion in pediatric cardiac surgery
Antifibrinolytics in pediatric cardiac surgery
MUF in pediatric cardiac surgery
Concentration of red cells and coagulation factors
Decreased red cell and coagulation factors
Decreased need for inotropic support
Shorter ventilator times
Shorter ICU stay
Shorter hospital stay
Other methods to minimize transfusion
Other hemostatic agents
Thrombosis in pediatric cardiac surgery
Risk factors for thrombosis in children with CHD
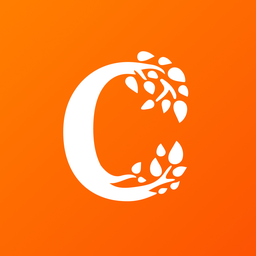
Full access? Get Clinical Tree
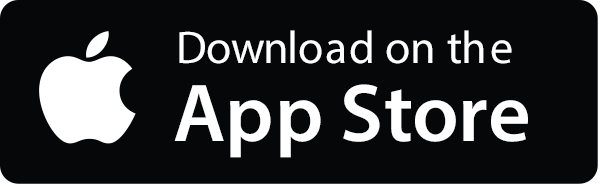
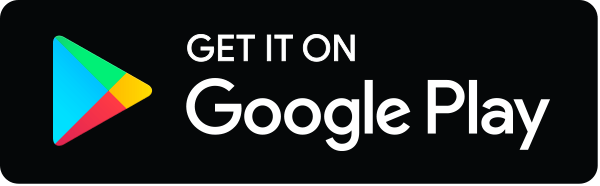