CHAPTER 12. Mechanical Ventilation
Carol Rhoades and Rose Linsler
The indications for endotracheal intubation are varied (Box 12-1). Once the airway is secured, the transport team must decide which method and mode of ventilation is most appropriate given the patient’s clinical scenario.
BOX 12-1
Get Clinical Tree app for offline access
Indications for Endotracheal Intubation
Problem: Failure or Anticipated Failure to Protect the Airway
• Obtunded or comatose with loss of gag reflex
• Traumatic brain injury
• Overdose
• Anoxia
• Cerebral insults (cerebrovascular accident [CVA], aneurysms, etc)
• Obstruction
• Edema related to trauma
• Inhalation injury
• Foreign body aspiration
• Congenital anomaly
• Pharmacologic therapy: Profound sedation or analgesia used to treat or diagnose certain conditions, such as:
• Status epilepticus
• Increased intracranial pressures
• Diagnostic procedures in combative patients
• Elective procedures
Problem: Failure to Oxygenate
• Shunt ventilation: Alveoli are perfused but not ventilated
• Pneumonia
• Acute lung injury (ALI)
• Pulmonary hemorrhage or contusion
• Atelectasis
• Congenital cardiac disease
• Dead space ventilation: Alveoli are ventilated but not perfused
• Pulmonary embolus
• Hypotension
• Low cardiac output states
• Diffusion abnormalities: Obstruction or restriction of gas exchange across the capillary-alveolar membrane
• Pulmonary edema
• Pulmonary fibrosis
• Oxygen extraction: Inability to extract oxygen
• Sepsis
• Carbon monoxide poisoning
• Cyanide poisoning
Problem: Failure to Ventilate
• Neurologic
• Spinal cord injury or disease
• Brain injury or disease
• Overdose
• Guillain-Barré syndrome
• Muscular
• Myopathies
• Myasthenia gravis
• Anatomic
• Pleural effusions
• Hemothorax or pneumothorax
• Abdominal compartment syndrome
• Bronchospasm, reactive airway disease
• Congenital anomalies
• Infectious
• Botulism
• Respiratory syncytial virus (RSV)
• Pertussis
Several advantages of mechanical ventilation over manual ventilation are found. Mechanical ventilation provides consistent and uninterrupted ventilation, which is of particular benefit in lengthy transports or in patients with minimal respiratory reserve in whom even a brief discontinuation of bag-mask ventilation may lead to desaturation and slow recovery. Ventilators also provide ventilatory parameter support that helps reduce lung injury and recruit alveoli in patients with acute lung injury (ALI) and acute respiratory distress syndrome (ARDS). In addition, patients with unstable conditions who need multiple interventions during transport are best served when all crewmembers are available to efficiently and quickly provide the care needed. The use of a ventilator eliminates the need for one crewmember to manually ventilate, thereby allowing all crewmembers to address other issues of care.
The advent of smaller, lighter, and more sophisticated ventilators extends the capability and flexibility available to the critical care transport provider. The type of ventilator chosen and its capabilities depend on mission profile, weight and space restrictions, and population served. Whichever ventilator is selected, medical transport personnel are challenged to understand the terminology (Table 12-1), modes, strategies, and complications of ventilation to proficiently set parameters and troubleshoot ventilation problems. A thorough understanding of blood gas analysis is a prerequisite to monitoring and adjusting ventilation parameters.
Term | Definition |
---|---|
Acute Lung Injury (ALI) | Pulmonary condition characterized by acute hypoxemic respiratory failure, diffuse bilateral pulmonary infiltrates on CXR, pulmonary wedge pressure < 18 mm Hg, and PaO 2/FiO 2 ratio of < 300. |
Adult respiratory distress syndrome (ARDS) | Severe form of ALI differentiated by a PaO 2/FiO 2 ratio < 200. |
Asynchrony | Incongruity between patient’s respiratory effort and ventilator breath delivery. Increases work of breathing. |
Auto-PEEP | Gas trapped in alveoli at end of expiration caused by insufficient expiration time, bronchospasm, or mucous plugging. Causes dynamic alveolar hyperinflation and increases work of breathing. Also referred to as intrinsic PEEP. |
Barotrauma | Damage to lung tissue from high airway pressures. Alveolar rupture may lead to pneumothorax, pulmonary interstitial edema, and pneumomediastinum. |
Cyclic atelectasis | Repeated opening of alveoli on inspiration and collapsing on expiration. |
Derecruitment | Collapse of open alveoli. |
Dynamic alveolar hyperinflation | Increase in lung volume at end of expiration caused by incomplete exhalation. |
Extrinsic PEEP | Mechanical application of PEEP. See PEEP. |
Fraction of inspired oxygen (FiO 2) | Fraction of inspired oxygen ranges from 0.21 (21%) to 1.0 (100%). Normal ambient air FiO 2 is 0.21. |
Functional residual capacity (FRC) | Volume of air remaining in lungs at end of normal expiration. |
Ideal body weight (IBW) | Expected weight of person based on gender and height. Used to base targeted tidal volume in adult population. |
Male | IBW = 50 kg + 2.3 kg for each inch over 5 ft. |
Female | IBW = 45.5 kg + 2.3 kg for each inch over 5 ft. |
I:E ratio | Ratio of inspiratory time to expiratory time. In normal conditions, expiratory phase is passive and twice as long as active inspiratory phase (1:2). |
Inspiratory flow | Rate in which breath is delivered on ventilator. It is measured in liters per minute. The higher the flow, the faster the breath is delivered. Flow is equal to tidal volume divided by inspiratory time. |
Inspiratory time (Ti) | Time over which tidal volume is delivered or pressure maintained (depending on mode). Set as I:E ratio or inspiratory flow. |
Intrinsic PEEP | See auto-PEEP. |
Mean Airway Pressure (P aw) | Average pressure to which lungs are exposed over one inspiratory/expiratory cycle. |
Minute ventilation (VE; MV) | Volume of air that moves in and out of lungs in 1 minute. It is product of tidal volume and respiratory rate. VE = Vt × rate. |
PaO 2/FiO 2 ratio | Calculation used to quantify to degree of hypoxemia and oxygenation abnormality in patients with acute respiratory failure. PaO 2 derived from arterial blood gas is divided by fraction of inspired oxygen. Normal is 500. Patients with ALI are at < 300, and patients with ARDS are at < 200. The lower the number, the greater the degree of pulmonary abnormality. For example, a patient on 60% FiO 2 has PaO 2 of 70 mm Hg: 70/0.6 = 115. By definition, this patient has ARDS. |
Peak inspiratory pressure (PIP) | Measurement in lungs at peak of inspiration. |
Permissive hypercapnia | Lung-protective ventilation strategy that uses low tidal volumes to reduce lung injury associated with high volumes and alveolar overdistention. Carbon dioxide is allowed to rise as consequence. |
Positive end-expiratory pressure (PEEP) | Positive pressure maintained at end of expiration. Therapy used in mechanical ventilation to increase volume of gas remaining in lungs at end of expiration (FRC). |
Plateau pressure | Pressure exerted on small airways and alveoli measured by holding inspiratory pause during ventilator delivered inspiration. Plateau pressures ≥ 30 mm Hg have been associated with alveolar overdistention lung injury. |
Recruitment | Refers to opening of collapsed alveoli. Alveolar recruitment maneuvers refer to increasing PEEP, for short durations, to open collapsed alveoli and improve oxygenation. Level of PEEP, duration, and frequency of this maneuver is determined by clinician. |
Tidal volume | Volume of gas inspired or expired in one breath. |
Trigger-sensitivity | Measure of amount of negative pressure that must be generated by patient to trigger mechanical ventilator into inspiratory phase. |
V/Q ratio | Ventilation (V) to perfusion (Q) ratio. |
High V/Q = deadspace ventilation. Alveoli are ventilated, but perfusion to lungs is impaired. Examples: pulmonary embolus, hypotension. | |
Low V/Q = shunt ventilation. Alveoli are perfused, but there is impaired aeration. Examples: ARDS, pneumonia. | |
Volutrauma | Volume-related overdistention injury of alveoli inflicted by mechanical ventilation. |
TRANSPORT VENTILATOR SELECTION
Multiple resources are available to programs researching the purchase of a transport ventilator. In the United States, the Food and Drug Administration (FDA) is the regulatory agency that must approve all medical equipment, including transport ventilators, before utilization in practice. Transport teams can also look to the American Society of Testing and Materials (ASTM) for established minimal specifications for safety and performance requirements on ventilators. 30 In addition, programs should verify airworthiness testing, which analyzes equipment for electromagnetic disturbances (radio transmission) and for functionality with exposure to vibration and environmental extremes.
Transport ventilators can be broadly classified as automatic resuscitators, simple, or sophisticated. 5Automatic resuscitators are rudimentary devices with minimal or no monitoring and alarm capabilities. The fractional concentration of oxygen in inspired gas (FiO 2) is generally fixed, and set parameters are limited to rate and tidal volume. They are intended for the prehospital setting, to be used by medical personnel with limited exposure to ventilation management. Examples include Ambu Matic® (Ambu, Copenhagen), Vortran Automatic Resucitators™ (VAR) Models RT and RC, (VORTRAN Medical Technologies, Sacramento, CA) and Oxylator® EM-100, EMX, or FR-300 (CPR Medical Devices, Inc, Ontario, Canada). Simple ventilators offer greater choices in mode of ventilation, rate, volume, and FiO 2. They offer demand flow for spontaneously breathing patients, more alarm features, and minimal monitoring options. Examples of simple ventilators include AutoVent® 2000 and 3000 (Allied Healthcare Products Inc, St Louis, MO), Uni-Vent® 706 (Impact® Instrumentation, Inc., West Caldwell, NJ), and Pneupac® paraPAC (Smiths Medical PM, Inc., Waukesha, WI). These ventilators are also intended for use in the prehospital setting. Sophisticated ventilators supply multiple modes of ventilation, for example, continuous positive airway pressure (CPAP), synchronized intermittent mandatory ventilation (SIMV), continuous mandatory ventilation (CMV), and flow-by and pressure support, to list a few. These ventilators are equipped with a full complement of alarms and monitoring capabilities. 5 Trained medical personnel proficient in ventilation management use them for interfacility transports. Examples of these sophisticated ventilators include Crossvent 3 or 4 (Bio-Med Devices, Inc., Guilford, CT), Oxylog® 2000 or 3000 (Dräger Medical, Telford, PA), LTV® 1200 (Viasys® Healthcare, Minneapolis, MN) and Bird® Avian (Bird Products Corporation, Palm Springs, CA).
Choice of ventilator varies with program profile. Some factors that should be considered follow.
Program Considerations
Mission Type
Whether a program transports adult, pediatric, or neonatal patients influences ventilator choices. Pediatric patients need ventilators capable of delivering lower tidal volumes. For example, a 10-pound (4.5-kg) 2 month-old ventilated with a tidal volume of 6 mL/kg needs a ventilator to deliver volumes as low as 27 mL. If the program transports critically ill newborn patients, a ventilator with high-frequency ventilation mode may be necessary. Programs that transport the full spectrum of patients from neonates to adults often need more than one type of ventilator.
Critical care transport teams need a more sophisticated ventilator as opposed to programs whose primary mission is scene flights. Patients with severe adult respiratory distress syndrome are a challenge to oxygenate and ventilate, even with highly sophisticated ventilators, and attempts to bag ventilate are likely to be unsuccessful. Teams that cover a large geographic area want to look at oxygen consumption and battery life of the ventilator.
Budget
In addition to the outright cost of the ventilator, consideration of clinical engineering availability for preventative maintenance and repairs should be weighed. Warranty coverage, loaner availability, manufacturer support, service record, and turnaround time for major repairs should also be explored.
Training
Mechanical ventilators require initial and on-going training. The more sophisticated the ventilator, the more training required. Highly sophisticated ventilators may require the addition of a respiratory therapist skilled in ventilation management. A transport program’s quality assurance team should monitor the ability (or inability) to adequately ventilate patients during transport and address educational needs.
Ventilator Considerations
Guidelines
The American Association of Respiratory Care (AARC) has established a consensus statement on the essentials of mechanical ventilators. 1 Components are classified as either essential or recommended (Table 12-2). Manufactures of sophisticated transport ventilators are guided by these recommendations.
As outlined in the Consensus Statement of the Essentials of Mechanical Ventilators from the American Association for Respiratory Care. | |
Essential | Recommended |
---|---|
Set Parameters: | Set Parameters: |
Positive pressure ventilation 100% FiO 2 PEEP Mandatory rate | Flow or I:E ratio Spontaneous breath modes Triggering mechanism |
Monitoring Capabilities: | Monitoring Capabilities: |
Peak airway pressure PEEP pressures | Expired tidal volume Expired spontaneous volume I:E ratio Mechanical rate Spontaneous rate |
Alarms: Level 1 (immediately life-threatening) | |
Power failure Loss of gas source Absence of gas delivery (apnea) Excessive gas delivery Exhalation valve failure Timing failure | |
Alarms: Level 2 (potentially life-threatening) | |
Battery power loss Circuit leak Blender failure Circuit occlusion Loss of or excessive PEEP Auto-cycling |
Durability and Safety
Transport ventilators should be compact, lightweight, and easy to secure; have proper electromagnetic shielding; and tolerate vibration and extremes of temperatures. Some monitor screens are difficult to see in the sunlight or dim lighting. Contrast adjustments should have a wide range. Alarms should have visual and loud audible alerts. Ventilator setting and alarm buttons or dials should not be easy to inadvertently reposition.
Oxygen Consumption
In addition to the patient-delivered gases, some ventilators use a gas source to drive the pneumatics, increasing the gas consumption of the ventilator during ventilation. A wide range of oxygen consumption exists between transport ventilators that should be compared with the transport range within a program.
Power
Battery life is of particular concern to programs that cover large geographic areas where transports occur over hours rather than minutes. Lithium ion batteries have one of the best energy-to-weight ratios and a longer shelf life as a result of a slower loss of charge when not in use and have no memory effect, which causes rechargeable batteries to lose charge capacity over time. They are also the most expensive. NiCad batteries are particularly susceptible to memory effect. Battery life on transport ventilators can range from less than 2 hours to 14 hours.
Selection Process
An organized selection process should be used in the search for a transport ventilator. Following is one suggested evaluation tool:
1. With the mission profile in mind, have the members of the flight team list and rank desired ventilator criteria. Indicate required components versus “nice-to-haves.” Is the team more comfortable with volume-controlled or pressure-controlled ventilators, or does the ventilator provide both options?
2. Recruit respiratory therapists and medical directors in the criteria listing process.
3. Gather a list of ventilators that best meet the criteria.
4. Rule out any ventilators on the list that do not meet airworthiness testing criteria or regulatory approval.
5. Search for published bench-testing reports.
6. Bring the ventilator into a clinical engineering bench test to verify that the ventilator functions as per published specifications. Some of the other considerations might be ease of use, safety, durability, portability, power usage, oxygen consumption, and functionality.
7. Use clinical engineering to assess for preventative maintenance, parts availability, and field repairs.
8. Use the ventilator on patients. If possible, trial it on patients in the hospital before field testing.
9. Review the data from steps 6, 7, and 8 against the criteria gathered in steps 1 and 2.
10. Rank the ventilators from most desired to least desired.
11. Use the program’s budgetary process to assist with financial negotiations.
12. The evaluation team makes the final recommendation on the basis of the team criteria, clinical use, and financials.
13. Make a recommendation to management.
VENTILATOR-INDUCED LUNG INJURY
Ventilator-induced lung injury (VILI) is damage done to the lung as a direct result of mechanical ventilation. Patients with acute lung injury, ARDS, chronic obstructive lung disease (COPD), and asthma are particularly prone to VILI associated with high transpulmonary pressures, high tidal volumes, and cyclic atelectasis, which compounds the difficulty in ventilation management. Transpulmonary pressure is the pressure difference across the lung calculated by subtracting the pleural pressure from the alveolar pressure. Alveolar pressure is most closely approximated in ventilated patients by the end-inspiratory plateau pressure (Pplat). The Pplat is believed to be a better indicator of alveolar overdistention than the peak inspiratory pressure (Ppk) because it is not influenced by upper airway resistance or ventilator equipment. 26 The crew measures the Pplat by pressing the inspiratory pause button for 0.5 to 1 second and taking a pressure reading.
Barotrauma
Barotrauma is the damage to lung tissue that causes alveolar rupture and migration of air into the extrapulmonary space. Historically, this has been attributed to high airway pressure. Barotrauma may lead to pneumothorax, tension pneumothorax, pneumomediastinum, air embolus (rare), and subcutaneous emphysema. Whether high Ppks are a direct cause of barotrauma or just a marker of severe lung disease is not clear. 16,34 In a retrospective study of patients enrolled in the ARDS Network trial of low-tidal volume ventilation, mean airway pressure and plateau pressure were not predictive indicators of barotrauma. 10
Volutrauma
More recently, attention has been placed on ventilator-induced injury caused by high volumes and alveolar overdistention, also referred to as dynamic hyperinflation. The stretch of alveoli causes microvascular injury, high permeability pulmonary edema, accumulation of fluid in the interstitial and alveolar space, disruption of surfactant function, and alveolar collapse. 9,22,24 Monitoring and controlling the Pplat is one strategy used to prevent or minimize the excessive alveolar stretch associated with VILI. The ARDS Network study demonstrated that maintenance of the Pplat at ≤30 cm H 2O was associated with a statistically significant decrease in ventilator days and improved mortality rates. 4 The Network ventilation protocols set a goal plateau pressure of ≤30 cm H 2O and recommend it be checked after each change in positive end-expiratory pressure (PEEP) or tidal volume. Some controversy exists regarding the need to reduce tidal volume if the Pplat is ≤30 cm H 2O; however, a secondary analysis of the ARDS trial suggested that a beneficial effect was seen in tidal volume reduction from 12 mL/kg ideal body weight (IBW) to 6 mL/kg regardless of the Pplat before the tidal volume was reduced. 17
Not only patients with ARDS/ALI are at risk for VILI with high volume ventilation. In a retrospective cohort study of 332 patients, 24% who did not have ALI at the initiation of mechanical ventilation developed injury within 5 days. 15 One of the primary risk factors for the development of ALI was high tidal volume (>9 mL/kg IBW) ventilation, which suggests that high tidal volumes may lead to the development of ALI in patients at risk.
Overdistention injury is not only associated with high tidal volumes. A normal tidal volume delivered to a diseased lung with large areas of low compliance can cause overdistention of available alveoli. Large segments of alveoli may be closed in patients with ARDS/ALI, leaving a small portion of the lung to receive the full tidal volume, which results in high pulmonary pressures and increasing susceptibility to volutrauma. 14
Cyclic Atelectasis
Cyclic atelectasis is the opening of alveoli on inspiration and collapsing on expiration. Animal models have shown that this repeated opening and closing causes the release of cytokines and development of local and systemic inflammatory response, 40 further extending lung injury. PEEP is used to curtail the collapsing of lung units on expiration, particularly in low tidal volume strategies.
Oxygen Toxicity
High levels of oxygen over a prolonged period of time have been shown to produce cytotoxic effects, presumably as the result of free radical production. 13 How this relates to the clinical care of patients with acute respiratory failure is unclear. Generally, transport times are of short enough duration not to produce the consequences of high oxygen levels in the adult or pediatric population. Administration of 100% oxygen to patients is reasonable during emergencies and resuscitation and for the duration of transport. Life-threatening hypoxia should always be treated with 100% oxygen delivered via facemask, endotracheal tube, or tracheotomy.
Some patients may be more susceptible to high levels of oxygen, for example, preterm neonates at risk for long-term retinal damage and bronchopulmonary dysplasia (BPD). Also susceptible are children with congenital cyanotic and partially repaired cyanotic-heart lesions who are at risk of hemodynamic instability as a result of oxygen-induced excess pulmonary blood flow. 38,43
CLASSIFICATION OF POSITIVE PRESSURE VENTILATION
No universal consensus exists on classification of ventilators or ventilation modes, and descriptions vary with the literature. The elements in classification that are most pertinent to transport teams are volume-targeted or pressure-targeted positive pressure ventilation and continuous positive airway pressure. No research exists to suggest improved outcomes between volume-controlled and pressure-controlled ventilation strategies. Within these categories, ventilation modes can be described as mandatory, assisted, or spontaneous, depending on the dynamic necessary to initiate an inspiratory breath.
▪ Mandatory: Mandatory breaths are initiated, controlled (volume or pressure), and terminated by the ventilator. No synchronizing of the ventilator breaths occurs with patient-initiated breaths. Example: CMV.
▪ Assisted: Spontaneous breaths are initiated by the patient but controlled and ended (assisted) by the ventilator. Examples: Assist-control and pressure support.
▪ Spontaneous: Spontaneous breaths are initiated, controlled, and terminated by the patient. Example: CPAP.
Volume-Targeted Ventilation
Volume-controlled pressure-variable ventilation is the most common mode of ventilation used on transport ventilators. The tidal volume is preset and delivered during the set inspiratory time of the ventilatory cycle. Once that tidal volume is reached, inspiration ends and exhalation begins. The inspiratory pressure varies depending on the compliance and resistance of the lung, with higher pressures associated with greater lung resistance or low compliance. The advantage of this form of ventilation is the guarantee of minute ventilation (tidal volume multiplied by rate). On the other hand, the potential for lung injury exists when high pressures are required to deliver the set tidal volume in patients with low lung compliance. To mitigate against ventilator-induced barotrauma or lung injury, pressure limits are set. If the limit is reached during the delivery of the set tidal volume, the inspiration is terminated, even if the targeted tidal volume has not been achieved. Modes of volume-cycled ventilation include CMV, assist-control (AC), and SIMV.
Continuous Mandatory Ventilation
Continuous mandatory ventilation (CMV) is a volume-cycled mode of ventilation in which the tidal volume and ventilatory rate are set. Spontaneous respiratory effort by the patient is ignored, and no patient triggering is possible. This method of ventilation is uncomfortable and is primarily used in sedated or chemically paralyzed patients who make no spontaneous respiratory effort. CMV-assist is a term used by some ventilator manufacturers to refer to assist-control ventilation.
Assist-Control Ventilation
With assist-control ventilation (AC), the clinician sets a base ventilatory rate; however, the patient is allowed to breathe faster than the set rate. Every breath, whether patient or ventilator initiated, receives the full set tidal volume. This mode of ventilation requires minimal work from the patient and is often used during the early hours and days after intubation to allow the patient to rest while the underlying cause of respiratory failure is addressed.
Assist-control can be either volume-controlled (ACV) or pressure-controlled (PCV). In volume-targeted assist-control, both the ventilatory rate and tidal volume are set parameters. Parameters also set by the clinician on more sophisticated transport ventilators include inspiratory flow, waveform, and trigger sensitivity. Patient hyperventilation may occur in this mode; therefore, minute ventilation should be monitored. Auto-PEEP can occur when respiratory rates are rapid and expiration is incomplete. The use of pop-off valves and pressure limit alarms prevents excessive peak inspiratory pressures in such cases, but the tidal volume delivered is diminished.
Some ventilators use the CMV term in referring to assist-control mode. With setting of a trigger sensitivity in CMV mode, the ventilator assists spontaneous respiratory efforts in the same manner described previously.
Synchronized Intermittent Mandatory Ventilation
Synchronized intermittent mandatory ventilation (SIMV) is a volume-controlled mode of ventilation. The primary distinction between SIMV and assist-control ventilation is how the ventilator contributes to spontaneous respiratory effort. Similar to AC, SIMV mode provides breaths at a set rate and set tidal volume. Unlike AC, spontaneous respiratory efforts do not receive an assisted tidal volume. Most modern ventilators synchronize a patient’s spontaneous efforts with the ventilator-delivered breath, preventing the delivery of a breath when the patient is already maximally inhaling or forcefully exhaling.
< div class='tao-gold-member'>
Only gold members can continue reading. Log In or Register a > to continue
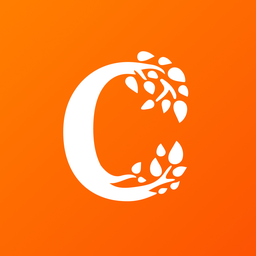
Full access? Get Clinical Tree
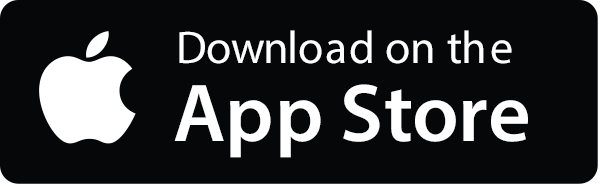
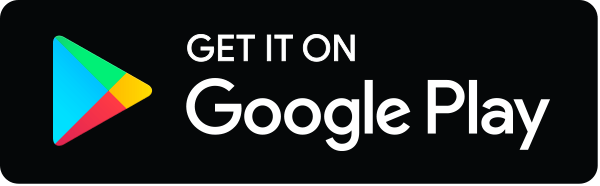
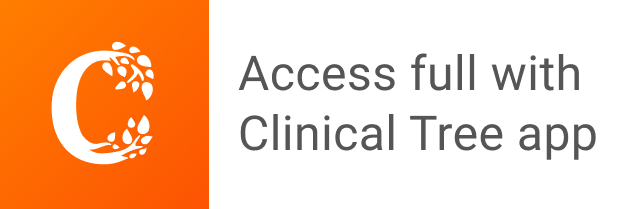