Chinedu G. Otu1, Dean B. Andropoulos2, and Emad B. Mossad1 1 Arthur S. Keats Division of Pediatric Cardiovascular Anesthesiology, Department of Anesthesiology, Texas Children’s Hospital, Baylor College of Medicine, Houston, TX, USA 2 Department of Anesthesiology, Perioperative and Pain Medicine, Department of Anesthesiology, Baylor College of Medicine, Texas Children’s Hospital, Houston, TX, USA A wide variety of anesthetic regimens are used for patients with congenital heart disease (CHD) undergoing cardiac or non‐cardiac surgery, procedures in the cardiac catheterization laboratory, or other diagnostic or therapeutic procedures such as magnetic resonance imaging (MRI). The goal of all of these regimens is to produce general anesthesia or adequate sedation while preserving systemic cardiac output (CO) and oxygen delivery. Many of these patients have a limited cardiac reserve, and if a cardiac arrest or other adverse cardiac event occurs, successful resuscitation is less frequent than in patients with normal hearts [1]. Thus, intelligent selection of regimen and dosage, with the patient’s unique pathophysiology in mind, along with anesthetic requirements for the particular procedure they are undergoing, is essential. This chapter reviews the effects on hemodynamics and myocardial contractility of anesthetic agents and muscle relaxants commonly used for patients with CHD. Although halothane is no longer available in the US, it is still used in some parts of the world, and it serves as the basis for comparison with newer agents because of the number of studies of its hemodynamic effects in children with and without heart disease. Therefore, data concerning halothane will be presented in this section. In vitro studies of effects on contractility in isolated adult human atrial fibers indicate that direct myocardial contractility depression is at its greatest with halothane and that sevoflurane is equal to isoflurane and desflurane [2] (Figure 10.1). These studies of myocardium reveal that the differences among these agents occur primarily from differing effects on calcium flux through L‐type Ca2+ channels, both transsarcolemmal and in the sarcoplasmic reticulum (SR). Halothane reduces Ca2+ flux through the sarcolemma more than isoflurane, with the net result of less intracellular Ca2+ available to bind to the troponin–actin–myosin complex, which produces myocyte contraction. Another mechanism of myocardial depression is that halothane, but not isoflurane, directly activates ryanodine‐sensitive SR Ca2+ channels, thereby reducing Ca2+ storage in the SR and making it less available for release during contraction. The effects of sevoflurane and desflurane on Ca2+ flux are similar to those of isoflurane [2]. It is important to note that infants from the newborn period up to an age of approximately 6 months exhibit an exaggerated degree of depression of myocardial contractility and blood pressure in response to all volatile agents, but especially halothane [3, 4] (Figure 10.2). This is probably due to the immaturity of the Ca2+ release and reuptake system, necessitating higher levels of free cytosolic Ca2+ to be available to bind to the troponin–actin–myosin complex to produce myocyte contraction [5]. Sevoflurane, and to a greater extent halothane, interferes with both L‐type Ca2+ channel and Na+/Ca2+ exchanger Ca2+ flux at the plasmalemmal membrane more in neonatal than in adult rat myocytes [6]. The volatile anesthetics interfered with Ca2+ release from the SR more in adult rat myocytes. Ca2+ release from the SR is thought to be less contributory to cytosolic Ca2+ levels in neonatal rat myocytes [7]. This information provides a mechanism for what is commonly observed clinically. Figure 10.1 Force of contraction over time (+dF/dt) of isolated adult human atrial trabeculae in response to 0–2.5 minimum alveolar concentration (MAC) anesthetics. Desflurane AB = desflurane in the presence of α‐ and ß‐receptor blockade. Halothane depresses contractility significantly more than all other agents at every MAC. (Source: Hanouz et al. [2]. Reproduced with permission of Wolters Kluwer Health, Inc.) The effects of volatile agents on systemic vascular resistance (SVR), as measured by arterial blood pressure, differ between agents. Ca2+ flux in the smooth muscles of arterioles is reduced by all of these agents, resulting in less resting tone, and thus lower blood pressure and vascular resistance. Halothane exhibits the most pronounced reduction of blood pressure, due to the combination of reduction in arterial tone, as well as the more pronounced depression of myocardial contractility. Isoflurane and sevoflurane lower blood pressure primarily through a reduction in SVR [8]. Sevoflurane has largely replaced halothane throughout most of the world for induction and maintenance of anesthesia. The Pediatric Perioperative Cardiac Arrest Registry data demonstrated a decrease in anesthetic medication‐related cardiac arrests, from 37% of the total in 1994–1997 to 18% in 1998–2004; the authors primarily attributed this decrease to the less frequent use of halothane, leading to fewer arrests, particularly in young infants [9]. In patients with CHD, several studies have been performed comparing newer agents with halothane. A study using transthoracic echocardiography comparing halothane, isoflurane, and sevoflurane [8] in 54 children with two‐ventricle CHD (Table 10.1, Figure 10.3) reported that 1 and 1.5 MAC (minimum alveolar concentration) halothane caused significant myocardial depression, resulting in a decrease in mean arterial pressure (MAP, decline of 22 and 35%, respectively), ejection fraction (EF, decrease of 15 and 20%, respectively) and CO (decrease of 17 and 21%, respectively) in patients aged 1 month to 13 years undergoing cardiac surgery. Sevoflurane maintained both CO and heart rate (HR) and had less profound hypotensive (MAP decrease of 13 and 20% at 1 and 1.5 MAC, respectively) and negative inotropic (EF preserved at 1 MAC, 11% decrease at 1.5 MAC) effects than halothane. Isoflurane, at concentrations as high as 1.5 MAC, preserved CO and EF, caused less suppression of MAP (22 and 25%) than halothane, increased HR (17 and 20%), and decreased SVR (20 and 22%). Table 10.1 Hemodynamic changes in response to four anesthetic regimens in 54 children with congenital heart disease with two ventricles Source: Rivenes et al. [8]. Reproduced with permission of Wolters Kluwer Health, Inc. All values are means ± SD. CI, systemic cardiac index; EF, ejection fraction; HR, heart rate; LVEDVI, left ventricular end‐diastolic volume index; MAP, mean arterial pressure; SF, shortening fraction; SVI, stroke volume index; SVRI, systemic vascular resistance index. a P < 0.05, one‐way analysis of variance (ANOVA), different from 0 minimum alveolar concentration (MAC) within the same anesthetic group. b P < 0.05, two‐way ANOVA, halothane vs. sevoflurane and fentanyl‐midazolam at 1 and 1.5 MAC. c P < 0.05, two‐way ANOVA, fentanyl–midazolam vs. halothane at 0 MAC. d P < 0.05, two‐way ANOVA, fentanyl–midazolam vs. halothane, sevoflurane, and isoflurane at 1.0 and 1.5 MAC. Figure 10.2 Force of contraction (N/cm2) in neonatal vs. adult rat ventricular trabecular muscle. Baseline force of contraction is greater in adult tissue, and both halothane and sevoflurane depress contractility more in the neonatal than in adult ventricular muscle. Halothane depresses contractility to a greater extent in both age groups. Panels (A and B) report raw data, while panels (C and D) express results as a percentage of baseline contractility. *Indicates a significant difference between ages (P < 0.05; n = 12 for controls, and n = 6 for all other groups). †Indicates a significant difference between control and halothane groups. ‡Indicates a significant difference between 1 and 2 minimum alveolar concentration (MAC) anesthetic. (Source: Prakash et al. [4]. Reproduced with permission of Wolters Kluwer Health, Inc.) Russell and colleagues [10] compared sevoflurane to halothane in the pre‐bypass period in 180 children with a variety of cardiac diagnoses, including 14 with single‐ventricle physiology and 40 with tetralogy of Fallot (TOF). The incidence of significant hypotension, bradycardia, and arrhythmia requiring drug treatment with atropine, phenylephrine, epinephrine, or ephedrine was higher with halothane (two events per patient vs. one with sevoflurane). Serum lactate also increased slightly with halothane. In a randomized crossover comparison study of 1 MAC isoflurane vs. sevoflurane, Dalal and colleagues studied 10 children with a variety of CHD, including both single‐ and two‐ventricle diseases [11]. Stroke volume, EF, and cardiac index were determined for the systemic ventricle using cardiac MRI techniques. There were no differences between agents for these parameters, or differences in MAP or HR in this small study. The effects of these agents on pulmonary (Qp) and systemic blood flow (Qs) in 30 biventricular patients with left‐to‐right shunts (secundum ASD, VSD) have also been assessed. Halothane, isoflurane, and sevoflurane did not change Qp:Qs as measured by echocardiography [12]. Wang and colleagues assessed differences in HR, cardiac rhythm, and blood pressure in 55 infants aged 2–12 months during 8% sevoflurane induction [13]. Twenty‐nine had increased pulmonary blood flow (PBF) (atrial septal defect [ASD], ventricular septal defect [VSD], patent ductus arteriosus [PDA]), and 26 had decreased PBF (TOF, pulmonic stenosis). The first 10 minutes of the anesthetic were assessed, and differences between groups compared. HR decreased from baseline in both groups, with a greater decrease in patients with decreased PBF. Blood pressure decreased significantly in the increased PBF group but was unchanged in the patients with decreased PBF. Junctional rhythm was observed in one patient in the increased PBF group. Patients with a single functional ventricle comprise an increasing proportion of patients undergoing anesthetics for both cardiac and non‐cardiac surgery, and studies of the hemodynamic effects of anesthetic agents are limited. Ikemba and colleagues [14] studied 30 infants with a single functional ventricle immediately before their bidirectional cavopulmonary connection, randomized to receive sevoflurane at 1 and 1.5 MAC or fentanyl/midazolam at equivalent doses. Myocardial performance index (MPI), a transthoracic echocardiographic measurement of combined systolic and diastolic ventricular function that can be applied to single‐ventricle patients, was not changed with any of these regimens when compared with baseline, indicating that either sevoflurane or fentanyl/midazolam can be used in this population to maintain hemodynamic stability. The effect of sevoflurane on systolic ventricular function has been studied in specific populations of CHD patients. Muyskens and colleagues [15] performed a retrospective review of patients with repaired Tetralogy of Fallot (rTOF) undergoing cardiac magnetic resonance imaging (CMR). After propensity score matching, the rTOF patients who required general anesthesia with maintenance sevoflurane (range 0.83–2.83%) were found to have a significant decrease in the CMR‐derived left ventricular ejection fraction (49 vs. 56%) and CMR‐derived right ventricular ejection fraction (41 vs. 48%). Elhoff and colleagues [16] assessed changes in ventricular function during 17 instances of sevoflurane‐based general anesthesia for pediatric heart transplant recipients undergoing cardiac catheterization with endomyocardial biopsies. A baseline, pre‐anesthetic echocardiogram was obtained. A significant decrease in ventricular function from baseline, as measured by repeat echocardiography was noted after attainment of a stable maintenance phase of general anesthesia with sevoflurane (MAC 0.7–1.0). There was, however, no consistent evidence of alteration of diastolic function under general anesthesia. In normal children, desflurane commonly produces tachycardia and hypertension during the induction phase, followed by a slight reduction in HR and systolic blood pressure during steady state at 1 MAC anesthetic level [17, 18]. There are no reports of its hemodynamic profile in patients with CHD. In a study of 47 children (mean age 12.8 years) undergoing electrophysiological study for supraventricular tachycardia (SVT), desflurane allowed for induction of the SVT in all patients and demonstrated no clinically important differences in any electrophysiologic measurement, as compared with a fentanyl‐based anesthetic [19]. The very low arrhythmogenic potential of desflurane has been demonstrated to be similar to that of isoflurane [20]. Some patients (6–12%) exposed to sevoflurane develop arrhythmias, mostly atrial or junctional [21, 22]. A study performed in infants (mean age 7.5 months) found that sevoflurane induction caused a 20% incidence of junctional bradycardia (less than 80 beats/min). Isoflurane, when utilized in children for electrophysiologic studies and radiofrequency ablation for SVT, does not affect sinoatrial or atrioventricular (AV) node conduction, and all arrhythmias were easily induced [23]. There are case reports of sevoflurane causing torsade de pointes in children with congenital long QT syndrome; this effect may be due to the increase in HR often seen with induction of anesthesia with this agent [24]. In a study of sevoflurane inhalation induction using electronic anesthesia data, Kraemer and colleagues [25] compared 209 children with Down syndrome with or without CHD with 268 healthy controls without Down syndrome or CHD. Fifty‐seven percent of the Down syndrome patients experienced age‐defined hypotension and bradycardia during the first 6 minutes of anesthesia, as compared with 12% of patients without Down syndrome or CHD (odds ratio, 9.56; 95% confidence interval 6.06–15.09). Bradycardia was not further characterized, i.e., sinus vs. junctional. Nogami and colleagues [26] describe a case of transient cardiac arrest in a 14‐year‐old Down syndrome patient with repaired ASD, VSD, and PDA during inhalational induction with 5% sevoflurane for a dental procedure. Severe bradycardia (HR <30 bpm) developed 1 minute after induction, followed by asystole. The mechanism for cardiac arrest was postulated to be secondary to autonomic cardiac dysfunction commonly seen in the Down syndrome population. Few studies to date have addressed the effects of the different anesthetics on an important group of pediatric patients with heart disease: patients with cardiomyopathy or significantly decreased systolic ventricular function. Volatile agents were commonly employed in two retrospective series of anesthesia in children with cardiomyopathy. Lynch and colleagues administered volatile agents in 26% of 236 inductions, and only four patients (1.7%) had cardiac arrest on induction; none of the four received volatile agents [27]. Kipps and colleagues reported a series of 33 children; 12% received volatile agents for induction and 64% received them for maintenance [28]. Although there were 15 patients with severe complications, defined as significant hypotension, arrhythmia, cardiac arrest, extracorporeal membrane oxygenation cannulation, or death, no conclusions could be drawn about any association with use of volatile agents. Rather, severe ventricular dysfunction (shortening fraction <16%) was the most important predictor of complications. Diastolic function with halothane and isoflurane has been studied in animal models of cardiomyopathy [29, 30]. The two agents differ, with halothane producing negative lusitropic effects, while isoflurane conserves or may even improve diastolic function. There are no reports measuring diastolic function in response to anesthetic agents in patients with CHD. Despite its ubiquitous use as an adjunct to anesthetic induction and maintenance in patients with CHD, information regarding the effect of N2O on hemodynamics in patients with CHD is very limited. Its use may be relatively contraindicated where increased FiO2 is employed, or where enlargement of enclosed air collections is possible, such as in any intracardiac or intrathoracic surgery. Reports of increased pulmonary vascular resistance (PVR), sympathetic stimulation, or significantly decreased CO in response to N2O in adult patients have not been substantiated in children with or without the cardiac disease [31, 32]. In infants and small children with normal hearts, Murray and colleagues found that addition of 30 and 60% N2O to 1 MAC halothane or isoflurane resulted in a decreased HR and cardiac index, without changing EF and stroke volume measured echocardiographically [33]. These authors also demonstrated that when 0.6 MAC halothane or isoflurane was substituted for 60% N2O during 0.9 MAC isoflurane or halothane anesthesia, HR, MAP, and cardiac index were unchanged [34]. In 14 patients with CHD recovering from surgery, Hickey and colleagues [35] administered 50% N2O and observed a decrease of 9% in HR, 12% in MAP, and 13% in systemic cardiac index. However, mean pulmonary artery pressure and PVR were not significantly changed in these well‐ventilated patients with a PaCO2 of 34–35, and pH of 7.47–7.49, even in patients with elevated PVR at baseline. This single report represents the total number of patients with CHD in which N2O administration has been carefully studied. Despite this paucity of information, extensive clinical experience has demonstrated N2O to be safe and effective, particularly as an adjunct to inhaled induction of anesthesia for congenital heart surgery. Fentanyl and sufentanil have been studied as a sole anesthetic in patients with CHD. Hickey, Hansen, and colleagues [36–38] provided the basis for this technique with a series of studies in neonates and infants less than 1 year of age undergoing complex repairs, ranging from the Norwood operation to complete repair of biventricular lesions. Fentanyl doses of 50–75 μg/kg, and sufentanil doses of 5–40 μg/kg, administered with pancuronium 0.1–0.15 mg/kg, provided excellent hemodynamic stability with minimal changes in HR and blood pressure throughout the surgery. The increase in pulmonary artery pressure and resistance in response to suctioning in infants recovering from cardiac surgery was eliminated with 25 μg/kg fentanyl. Moore and colleagues [39] demonstrated that 5, 10, or 20 μg/kg sufentanil in children aged 4–12 years had no effect on EF as measured by echocardiography in patients undergoing repair of biventricular lesions. Increases in HR, blood pressure, and stress hormones were more effectively blunted by the higher doses. Glenski and colleagues [40] reported M‐mode echocardiographic measures of contractility, blood pressure, and HR response using fentanyl (100 μg/kg) or sufentanil (20 μg/kg) in children aged 6 months to 9 years. Measurements were made at three different times: after a premedication with morphine and scopolamine, after induction, and after tracheal intubation. These opioids decreased both EF and shortening fraction after induction, but they returned to or above baseline after intubation. Midazolam is often added to fentanyl anesthesia to provide sedation and amnesia, as a substitute for low‐dose volatile anesthetic agent, particularly in hemodynamically unstable patients and young infants, where the myocardial depressant effects of volatile agents are more pronounced. Fentanyl and midazolam combinations have been studied in two different clinically utilized dose regimens to simulate 1 and 1.5 MAC of volatile agents (fentanyl 8–18 μg bolus followed by 1.7–4.3 μg/kg/h infusion, then repeat bolus at 50% of the original doses followed by increase of infusion by 50%, depending on age; midazolam 0.29 mg/kg bolus followed by 139 μg/kg/h infusion, then repeat bolus 50% of the original dose, followed by increase in infusion of 50% for all ages) for induction and the pre‐bypass period in congenital heart surgery in biventricular patients [8] (Figure 10.3). Vecuronium was used for muscle relaxation in order to isolate the effects of the other two agents on hemodynamics. Measurements of CO and contractility were made by echocardiography. Fentanyl/midazolam caused a significant decrease (22%) in CO despite preservation of contractility. This was predominantly due to a decrease in HR. Co‐administration of a vagolytic agent such as atropine [41] or pancuronium would probably preserve CO. The added effect of midazolam on echocardiographic indices of contractility has not been previously reported, however, increased inotropic support requirements have been documented in infants receiving sedation after cardiac surgery with the addition of midazolam bolus totaling 0.3 mg/kg, and infusion of 0.1–0.2 mg/kg/h in the early postoperative period [42]. Figure 10.3 Hemodynamic changes assessed by echocardiography in 54 patients with two‐ventricle congenital heart disease. (A) Ejection fraction. (B) Cardiac output. H, halothane; F/M, fentanyl/midazolam; I, isoflurane; MAC, minimum alveolar concentration; S, sevoflurane. See text for details. (Source: Rivenes et al. [8]. Reproduced with permission of Wolters Kluwer Health, Inc.) The stress response to major cardiac surgery in infants and children has been the subject of considerable interest. Anand and Hickey reported the use of high dose sufentanil at a total mean dose of 37 μg/kg as the sole anesthetic for complex neonatal surgery [43]. The sufentanil was continued by infusion for 24 hours postoperatively. This regimen was compared with halothane plus morphine (mean dose of 0.35 mg/kg) intraoperatively, followed by intermittent morphine and diazepam postoperatively. Stress response, as measured by changes in adrenal hormones, cortisol, glucose, and lactate, was significantly reduced in the sufentanil group, and mortality and major complications such as sepsis and necrotizing enterocolitis were also significantly reduced. A later study from the same institution of 45 infants averaging 3 months of age undergoing biventricular repair with deep hypothermic cardiopulmonary bypass has been reported [44]. A total fentanyl dose of 100 μg/kg was administered, given either as intermittent boluses of 25 μg/kg, or as boluses plus infusion, with or without midazolam, and all regimens resulted in a significant endocrine stress response to cardiac surgery. Despite this, the outcome was excellent in all groups, with no adverse outcomes related to the anesthetic technique or the surgical stress response. The sole hemodynamic difference between the regimens was a lower MAP during cooling on bypass in the group who received midazolam. Finally, Duncan and colleagues [45] reported a dose–response study of 2, 25, 50, 100, and 150 μg/kg fentanyl before bypass in 40 children averaging 13 months and 8.5 kg. The 2 μg/kg group had significant increases in pre‐bypass norepinephrine, glucose, and cortisol, and significantly higher HR and blood pressure than all other groups. A dose of 25 μg/kg or higher eliminated changes in these parameters for the duration of the surgery. It is difficult to interpret the significance of these stress response studies because they were evaluated by different age groups and lesions. Also, there was more than one decade between reports, during which time there were significant improvements in surgical, bypass, and postoperative management. If any group of patients had benefited from attenuation of the stress response, it would appear to be neonatal patients undergoing complex surgery. Current approaches to early extubation favor lower intraoperative opioid doses, e.g., <10–20 μg/kg fentanyl and use of adjuvants such as dexmedetomidine or regional anesthesia, rather than completely ablating the stress response [46]. Chapter 24 presents an extensive discussion of early extubation approaches for congenital cardiac surgery. Remifentanil is a synthetic ultra‐short‐acting narcotic agent metabolized by plasma esterases with a half‐life of 3–5 minutes that is independent of the duration of infusion [47]. It is particularly useful for short non‐cardiac procedures with intense stimulation where opioid‐based anesthesia and its hemodynamic stability would be desirable, yet where rapid emergence is also important. Donmez and colleagues [48] reported a series of 55 children undergoing cardiac catheterization with a remifentanil infusion of 0.1 μg/kg/min. This regimen maintained excellent cardiovascular stability, with minimal changes in HR, blood pressure, or oxygen saturation. Fifty‐eight percent of patients required additional sedation with midazolam or ketamine. Apnea was infrequent, and a time to recovery score of 5 (10‐point scale) was only 2–4 minutes. Patients undergoing long cardiac catheterization procedures could potentially benefit from this agent. Remifentanil infusion at 0.3 μg/kg/min increased sinus cycle and Wenckebach cycle length from baseline but did not affect atrial‐His or His‐ventricular interval, or AV node, atrial, ventricular, or accessory pathway effective refractory period in 14 patients undergoing electrophysiology study and ablation for SVT [49]. In a similar study of 29 patients receiving 0.2 or 0.4 μg/kg/min remifentanil, the larger dose prolonged sinoatrial conduction time and sinus node recovery time, but not atrial‐His interval [50]. These electrophysiological effects should be taken into account if the drug is used for electrophysiological studies. Remifentanil administration has been reported for ASD repair, where patients are extubated in the operating room (OR) [51]. It apparently does not bind to the cardiopulmonary bypass (CPB) circuit [52] and its clearance in children before and after CPB appears to be predictable within a narrow range, making it a potentially useful agent for “fast‐track” anesthesia and early extubation for simple surgical procedures. Friesen and colleagues compared remifentanil 0.3–0.7 μg/kg/min with fentanyl 15 μg/kg, both with isoflurane and pancuronium, in fast‐track pediatric cardiac operations (ASD and VSD repairs) and found that HR was significantly slower in the OR in the remifentanil group, but there was no difference in time to extubation, analgesic requirements in ICU, nausea/vomiting or hypertension in ICU, or in ICU length of stay [53]. Akpek and colleagues compared higher‐dose remifentanil (2 μg/kg load and 2 μg/kg/min maintenance infusion) with fentanyl (20 μg/kg load and 20 μg/kg/h infusion) in 33 infants with pulmonary hypertension undergoing surgery for repair of left‐to‐right shunting defects. Both groups had a midazolam infusion. There were no clinically important differences in hemodynamic, respiratory, or oxygen saturation parameters between groups, and no difference in clinical outcomes [54]. Thus, despite some theoretical advantages due to its pharmacokinetic profile, there are few clinically significant differences between remifentanil and fentanyl. Propofol has become a popular agent for sedation for cardiac catheterization procedures and induction of general anesthesia for cardiac surgery. In plasma concentrations found in routine clinical use, propofol has minimal negative inotropic effects in isolated animal cardiac preparations [55] or in human adult atrial muscle strips [56]. In children with normal hearts, propofol at induction doses consistently decreases systolic and mean arterial pressure by 5–25% without changing HR [57]. In a case series of 13 preterm neonates of 29–32 weeks gestational age, a propofol bolus of 1 mg/kg decreased MAP from 38 to 24 mmHg, and 5 of 13 patients had a severe decrease to <25 mmHg [58]. There has been one published study using echocardiography to assess myocardial contractility and CO in infants with normal hearts induced with propofol [57]. The shortening fraction or cardiac index was not changed, and SVR decreased by 14 and 27% at 1 and 5 minutes after induction, respectively. Load independent measures of contractility (stress velocity index and stress shortening index) decreased significantly from baseline at 5 minutes after induction with propofol. Williams and colleagues [59] measured the hemodynamic effects of propofol in 31 patients aged 3 months to 12 years at a dose of 50–200 μg/kg/min undergoing cardiac catheterization (Figure 10.4). They found that propofol significantly decreased MAP and SVR; however, there was no change in systemic CO, HR, mean pulmonary artery pressure, or PVR. In patients with cardiac shunts, the net result was a significant increase in the right‐to‐left shunt, a decrease in the left‐to‐right shunt, and decreased Qp : Qs, resulting in a statistically significant decrease in PaO2 and SaO2, as well as reversal of the shunt from left‐to‐right to right‐to‐left in two patients. In another study of patients undergoing cardiac catheterization, Lebovic and colleagues [60] demonstrated that patients could experience a 20% decrease in HR or MAP. Combining propofol infusion with ketamine infusion for cardiac catheterization procedures demonstrated less change in MAP, preservation of baseline HR, and little effect on recovery time [61]. Although propofol is very useful for cardiac catheterization, short, stimulating procedures, and possibly for short‐term sedation after cardiac surgery, its long‐term use as an ICU sedative is contraindicated, with several reports of otherwise unexplained metabolic acidosis and myocardial failure after long‐term (>48 hours), high dose use in pediatric patients [64, 65]. The mechanism of this cardiovascular collapse is postulated to be due to disruption of fatty acid oxidation caused by impaired entry of long‐chain acylcarnitine esters into the mitochondria and failure of the mitochondrial respiratory chain [66]. Although a recent case series of short‐term, low‐ to medium‐dose post‐anesthetic propofol infusion (median 7 hours) has been described without complication in 12 high‐risk CHD patients with pulmonary hypertension, airway malacia, or underlying chromosomal abnormality, this practice should be limited to those centers with substantial expertise and experience with this technique [67]. Propofol infusions of duration greater than 6 hours, as might be observed for long cardiac catheterization procedures, are not recommended because of the potential for the propofol infusion syndrome. Propofol has no significant effect on sinoatrial or AV node conduction, or on the ability to induce SVT, and therefore is desirable as a primary agent during electrophysiologic studies and radiofrequency ablation [23, 62]. However, ectopic atrial tachycardia may be suppressed by propofol [63]. In summary, propofol can be utilized in patients with adequate cardiovascular reserve who can tolerate a mild decrease in contractility and HR, and a decrease in SVR. Propofol may cause an increased intracardiac right‐to‐left shunt, and reversal of shunt in some patients, (i.e. acyanotic TOF), and thus hemodynamic data obtained in the cardiac catheterization laboratory should be interpreted accordingly. Although propofol use for induction of anesthesia has been described for patients with cardiomyopathies in several case series [27, 28], many authorities recommend against its use, particularly in hypertrophic or dilated cardiomyopathy, where even a small reduction in afterload and preload from propofol’s venodilatory properties can result in cardiovascular collapse [68].
CHAPTER 10
Anesthetic Agents and Their Cardiovascular Effects
Introduction
Volatile agents
Measured and calculated hemodynamic and echocardiographic variables
Agent
MAC
HR (beats/min)
(mmHg)
EF (%)
SF (%)
SVI (mL/m2)
LVEDVI (mL/m2)
CI (L/min/m2)
SVRI (dyn s/cm5/m2)
Halothane
0
129 ± 22
77 ± 15
63 ± 9
40 ± 5
36 ± 16
44 ± 19
4.49 ± 1.87
1425 ± 622
1
130 ± 19
60 ± 11a
54 ± 12a
32 ± 7a,b
28 ± 11a
38 ± 14
3.47 ± 1.17
1331 ± 529
1.5
129 ± 17
49 ± 12a
50 ± 13a
30 ± 8a ,b
26 ± 11a
39 ± 12
3.34 ± 1.36a
1132 ± 503a
Sevoflurane
0
123 ± 32
67 ± 8
68 ± 11
44 ± 7
56 ± 41
37 ± 15
6.91 ± 4.32
1014 ± 653
1
126 ± 26
58 ± 13a
62 ± 9
39 ± 7
52 ± 31
36 ± 18
6.59 ± 4.04
883 ± 592
1.5
128 ± 25
58 ± 13a
58 ± 10a
39 ± 9
46 ± 26
35 ± 14
5.78 ± 3.06
782 ± 390
Isoflurane
0
112 ± 27
69 ± 12
63 ± 7
39 ± 5
46 ± 2
46 ± 24
4.96 ± 2.74
1377 ± 809
1
125 ± 16a
54 ± 9a
62 ± 8
37 ± 4
39 ± 17
40 ± 17
4.82 ± 2.20
1022 ± 601a
1.5
128 ± 13a
50 ± 9a
59 ± 9
36 ± 5
39 ± 17
42 ± 19
4.59 + 2.12
950 ± 513a
Fentanyl–midazolam
0
106 ± 22c
66 ± 8
63 ± 6
40 ± 6
46 ± 34
54 ± 25
5.16 ± 4.39
1261 ± 644
1
87 ± 19a,d
59 ± 11a
60 ± 7
39 ± 5
42 ± 30
47 ± 25
3.79 ± 3.05a
1540 ± 806
1.5
82 ± 18a,d
56 ± 11a
59 ± 7
38 ± 7
43 ± 30
52 ± 24
3.67 ± 2.99a
1559 ± 875
Nitrous oxide (N2O)
Opioids and benzodiazepines
Propofol
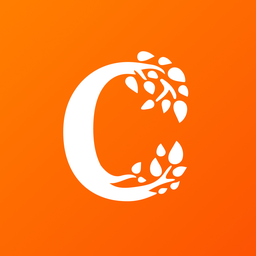
Full access? Get Clinical Tree
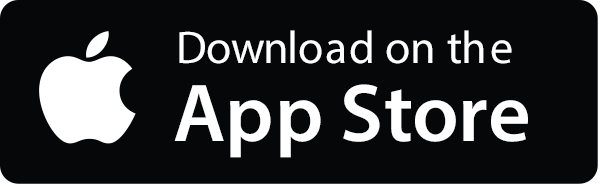
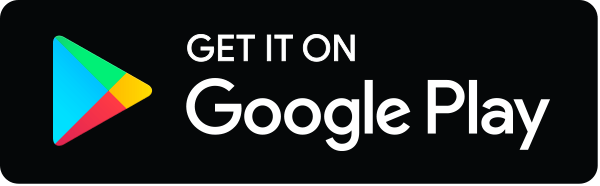